Organic Heterojunction Devices Based on Phthalocyanines: A New Approach to Gas Chemosensing
Abstract
: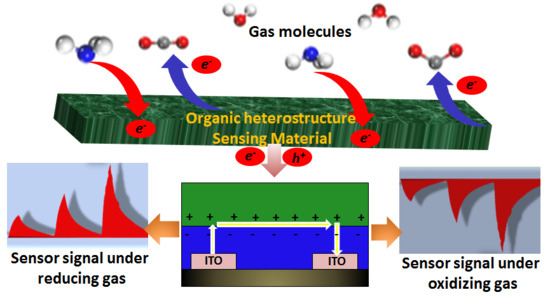
1. Introduction
2. Suitability of Phthalocyanines in Heterostructure Based Gas Sensors
2.1. Working Principles and Metrological Parameters
2.2. Metal Phthalocyanines in Organic Heterostructures
3. Organic Heterojunction Effects and Chemosensing Devices
3.1. Interfacial Energy Levels Alignment and Charge Distribution
3.2. MPc Heterostructure Integration in Chemosensing Devices
4. Chemosensing Properties of MPc-Based Heterostructures
4.1. OFET Based Gas Sensors
4.2. MSDI Based Gas Sensors
5. Conclusions and Outlook
Author Contributions
Funding
Acknowledgments
Conflicts of Interest
References
- Zou, S.-J.; Shen, Y.; Xie, F.-M.; Chen, J.-D.; Li, Y.-Q.; Tang, J. Recent advances in organic light-emitting diodes: Toward smart lighting and displays. Mater. Chem. Front. 2020, 4, 788–820. [Google Scholar] [CrossRef]
- Hains, A.W.; Liang, Z.; Woodhouse, M.A.; Gregg, B.A. Molecular Semiconductors in Organic Photovoltaic Cells. Chem. Rev. 2010, 110, 6689–6735. [Google Scholar] [CrossRef] [PubMed]
- Quinn, J.T.E.; Zhu, J.; Li, X.; Wang, J.; Li, Y. Recent progress in the development of n-type organic semiconductors for organic field effect transistors. J. Mater. Chem. C 2017, 5, 8654–8681. [Google Scholar] [CrossRef]
- Samuel, I.D.W.; Turnbull, G.A. Organic Semiconductor Lasers. Chem. Rev. 2007, 107, 1272–1295. [Google Scholar] [CrossRef] [PubMed]
- Heremans, P.; Gelinck, G.H.; Müller, R.; Baeg, K.-J.; Kim, D.-Y.; Noh, Y.-Y. Polymer and Organic Nonvolatile Memory Devices†. Chem. Mater. 2011, 23, 341–358. [Google Scholar] [CrossRef]
- Ray, A.K. Organic Materials for Chemical Sensing. In Springer Handbook of Electronic and Photonic Materials; Kasap, S., Capper, P., Eds.; Springer International Publishing: Cham, Switzerland, 2017; p. 1. [Google Scholar]
- Xue, R.; Zhang, J.; Li, Y.; Li, Y. Organic Solar Cell Materials toward Commercialization. Small 2018, 14, 1801793. [Google Scholar] [CrossRef]
- Song, R.; Wang, Z.; Zhou, X.; Huang, L.; Chi, L. Gas-Sensing Performance and Operation Mechanism of Organic π-Conjugated Materials. ChemPlusChem 2019, 84, 1222–1234. [Google Scholar] [CrossRef]
- Potje-Kamloth, K. Semiconductor Junction Gas Sensors. Chem. Rev. 2008, 108, 367–399. [Google Scholar] [CrossRef]
- Janata, J.; Josowicz, M. Chemical Modulation of Work Function as a Transduction Mechanism for Chemical Sensors. Acc. Chem. Res. 1998, 31, 241–248. [Google Scholar] [CrossRef]
- Oprea, A.; Simón, E.; Fleischer, M.; Frerichs, H.-P.; Wilbertz, C.; Lehmann, M.; Weimar, U. Flip-chip suspended gate field effect transistors for ammonia detection. Sens. Actuators B 2005, 111, 582–586. [Google Scholar] [CrossRef]
- Zhang, C.; Chen, P.; Hu, W. Organic field-effect transistor-based gas sensors. Chem. Soc. Rev. 2015, 44, 2087–2107. [Google Scholar] [CrossRef] [PubMed] [Green Version]
- Rani, V.; Sharma, A.; Kumar, P.; Singh, B.; Ghosh, S. Charge transport mechanism in copper phthalocyanine thin films with and without traps. RSC Adv. 2017, 7, 54911–54919. [Google Scholar] [CrossRef] [Green Version]
- Mateos, M.; Meunier-Prest, R.; Suisse, J.-M.; Bouvet, M. Modulation of the organic heterojunction behavior, from electrografting to enhanced sensing properties. Sens. Actuators B 2019, 299, 126968. [Google Scholar] [CrossRef] [Green Version]
- Mateos, M.; Tchangaï, M.-D.; Meunier-Prest, R.; Heintz, O.; Herbst, F.; Suisse, J.-M.; Bouvet, M. Low Conductive Electrodeposited Poly(2,5-dimethoxyaniline) as a Key Material in a Double Lateral Heterojunction, for Sub-ppm Ammonia Sensing in Humid Atmosphere. ACS Sens. 2019, 4, 740–747. [Google Scholar] [CrossRef] [PubMed] [Green Version]
- Bouvet, M.; Mateos, M.; Wannebroucq, A.; Navarrete, E.; Llobet, E. A tungsten oxide–lutetium bisphthalocyanine n–p–n heterojunction: From nanomaterials to a new transducer for chemo-sensing. J. Mater. Chem. C 2019, 7, 6448–6455. [Google Scholar] [CrossRef]
- Wu, Y.; Ma, P.; Wu, N.; Kong, X.; Bouvet, M.; Li, X.; Chen, Y.; Jiang, J. Two-Step Solution-Processed Two-Component Bilayer Phthalocyaninato Copper-Based Heterojunctions with Interesting Ambipolar Organic Transiting and Ethanol-Sensing Properties. Adv. Mater. Interfaces 2016, 3, 1600253. [Google Scholar] [CrossRef]
- Yan, D.; Wang, H.; Du, B. Interfacial Electronic Structure in Organic Semiconductor Heterojunctions. In Introduction to Organic Semiconductor Heterojunctions; Wiley: Hoboken, NJ, USA, 2010; pp. 87–123. [Google Scholar]
- Parra, V.; Bouvet, M. Semiconductor Tranducer and Its Use in a Sensor for Detecting Electron-donor or Electron-Acceptor Species. U.S. Patent 8,450,725 B2, 28 May 2013. [Google Scholar]
- Parra, V.; Brunet, J.; Pauly, A.; Bouvet, M. Molecular semiconductor-doped insulator (MSDI) heterojunctions: An alternative transducer for gas chemosensing. Analyst 2009, 134, 1776. [Google Scholar] [CrossRef]
- Bouvet, M. Radical Phthalocyanines and Intrinsic Semiconduction. In The Porphyrin Handbook; Kadish, K.M., Smith, K.M., Guilard, R., Eds.; Academic Press: Cambridge, MA, USA, 2003; Volume 19, pp. 37–103. [Google Scholar]
- Wong, Y.C.; Ang, B.C.; Haseeb, A.; Baharuddin, A.A.; Wong, Y. Review—Conducting Polymers as Chemiresistive Gas Sensing Materials: A Review. J. Electrochem. Soc. 2019, 167, 037503. [Google Scholar] [CrossRef]
- Miasik, J.J.; Hooper, A.; Tofield, B.C. Conducting polymer gas sensors. J. Chem. Soc. Faraday Trans. 1 1986, 82, 1117–1126. [Google Scholar] [CrossRef]
- Ozturk, Z.Z.; Kilinc, N.; Atilla, D.; Gürek, A.G.; Ahsen, V. Recent studies chemical sensors based on phthalocyanines. J. Porphyrins Phthalocyanines 2009, 13, 1179–1187. [Google Scholar] [CrossRef]
- Basova, T.V.; Ray, A.K. Review—Hybrid Materials Based on Phthalocyanines and Metal Nanoparticles for Chemiresistive and Electrochemical Sensors: A Mini-Review. ECS J. Solid State Sci. Technol. 2020, 9, 061001. [Google Scholar] [CrossRef]
- Gounden, D.; Nombona, N.; Van Zyl, W.E. Recent advances in phthalocyanines for chemical sensor, non-linear optics (NLO) and energy storage applications. Coord. Chem. Rev. 2020, 420, 213359. [Google Scholar] [CrossRef]
- Zagal, J.H.; Griveau, S.; Silva, J.F.; Nyokong, T.; Bedioui, F. Metallophthalocyanine-based molecular materials as catalysts for electrochemical reactions. Coord. Chem. Rev. 2010, 254, 2755–2791. [Google Scholar] [CrossRef]
- Wang, H.; Yan, D. Organic heterostructures in organic field-effect transistors. NPG Asia Mater. 2010, 2, 69–78. [Google Scholar] [CrossRef] [Green Version]
- Torsi, L.; Magliulo, M.; Manoli, K.; Palazzo, G. Organic field-effect transistor sensors: A tutorial review. Chem. Soc. Rev. 2013, 42, 8612. [Google Scholar] [CrossRef] [PubMed]
- Janata, J. Introduction to Sensors. In Principles of Chemical Sensors; Janata, J., Ed.; Springer: Boston, MA, USA, 2009; pp. 1–11. [Google Scholar]
- Grobosch, M.; Schmidt, C.; Kraus, R.; Knupfer, M. Electronic properties of transition metal phthalocyanines: The impact of the central metal atom (d5–d10). Org. Electron. 2010, 11, 1483–1488. [Google Scholar] [CrossRef]
- Nalwa, H.S. The effect of central metal atom on the electrical properties of phthalocyanine macromolecule. J. Electron. Mater. 1988, 17, 291–295. [Google Scholar] [CrossRef]
- Liao, M.-S.; Scheiner, S. Electronic structure and bonding in metal phthalocyanines, Metal=Fe, Co, Ni, Cu, Zn, Mg. J. Chem. Phys. 2001, 114, 9780–9791. [Google Scholar] [CrossRef] [Green Version]
- Davidson, A.T. The effect of the metal atom on the absorption spectra of phthalocyanine films. J. Chem. Phys. 1982, 77, 168. [Google Scholar] [CrossRef]
- Weiss, R.; Fischer, J. Lanthanide Phthalocyanine Complexes. In The Porphyrin Handbook; Kadish, K.M., Smith, K.M., Guilard, R., Eds.; Academic Press: Amsterdam, The Netherlands, 2003; Volume 16, pp. 171–246. [Google Scholar]
- Nyokong, T. Effects of substituents on the photochemical and photophysical properties of main group metal phthalocyanines. Coord. Chem. Rev. 2007, 251, 1707–1722. [Google Scholar] [CrossRef]
- Jones, T.; Bott, B.; Thorpe, S. Fast response metal phthalocynanine-based gas sensors. Sens. Actuators 1989, 17, 467–474. [Google Scholar] [CrossRef]
- Melville, O.A.; Lessard, B.H.; Bender, T.P. Phthalocyanine-Based Organic Thin-Film Transistors: A Review of Recent Advances. ACS Appl. Mater. Interfaces 2015, 7, 13105–13118. [Google Scholar] [CrossRef] [PubMed]
- Bouvet, M. Phthalocyanine-based field-effect transistors as gas sensors. Anal. Bioanal. Chem. 2005, 384, 366–373. [Google Scholar] [CrossRef] [PubMed] [Green Version]
- Bohrer, F.I.; Colesniuc, C.N.; Park, J.; Ruidíaz, M.E.; Schuller, I.K.; Kummel, A.C.; Trogler, W.C. Comparative Gas Sensing in Cobalt, Nickel, Copper, Zinc, and Metal-Free Phthalocyanine Chemiresistors. J. Am. Chem. Soc. 2009, 131, 478–485. [Google Scholar] [CrossRef]
- Bohrer, F.I.; Colesniuc, C.N.; Park, J.; Schuller, I.K.; Kummel, A.C.; Trogler, W.C. Selective Detection of Vapor Phase Hydrogen Peroxide with Phthalocyanine Chemiresistors. J. Am. Chem. Soc. 2008, 130, 3712–3713. [Google Scholar] [CrossRef]
- Park, J.H.; Royer, J.E.; Chagarov, E.; Kaufman-Osborn, T.; Edmonds, M.; Kent, T.; Lee, S.; Trogler, W.C.; Kummel, A.C. Atomic Imaging of the Irreversible Sensing Mechanism of NO2 Adsorption on Copper Phthalocyanine. J. Am. Chem. Soc. 2013, 135, 14600–14609. [Google Scholar] [CrossRef]
- Brunet, J.; Garcia, V.P.; Pauly, A.; Varenne, C.; Lauron, B. An optimised gas sensor microsystem for accurate and real-time measurement of nitrogen dioxide at ppb level. Sens. Actuators B Chem. 2008, 134, 632–639. [Google Scholar] [CrossRef]
- Ji, S.; Wang, H.; Wang, T.; Yan, D. A High-Performance Room-Temperature NO2Sensor Based on an Ultrathin Heterojunction Film. Adv. Mater. 2013, 25, 1755–1760. [Google Scholar] [CrossRef]
- Shaymurat, T.; Tang, Q.; Tong, Y.; Dong, L.; Liu, Y.C. Gas Dielectric Transistor of CuPc Single Crystalline Nanowire for SO2Detection Down to Sub-ppm Levels at Room Temperature. Adv. Mater. 2013, 25, 2269–2273. [Google Scholar] [CrossRef]
- Zhao, Y.; Guo, Y.; Liu, Y. 25th Anniversary Article: Recent Advances in n-Type and Ambipolar Organic Field-Effect Transistors. Adv. Mater. 2013, 25, 5372–5391. [Google Scholar] [CrossRef]
- Bouvet, M.; Gaudillat, P.; Kumar, A.; Sauerwald, T.; Schüler, M.; Schütze, A.; Suisse, J.-M. Revisiting the electronic properties of Molecular Semiconductor—Doped Insulator (MSDI) heterojunctions through impedance and chemosensing studies. Org. Electron. 2015, 26, 345–354. [Google Scholar] [CrossRef]
- Murdey, R.; Sato, N.; Bouvet, M. Frontier Electronic Structures in Fluorinated Copper Phthalocyanine Thin Films Studied Using Ultraviolet and Inverse Photoemission Spectroscopies. Mol. Cryst. Liq. Cryst. 2006, 455, 211–218. [Google Scholar] [CrossRef]
- Brinkmann, H.; Kelting, C.; Makarov, S.; Tsaryova, O.; Schnurpfeil, G.; Wöhrle, D.; Schlettwein, D. Fluorinated phthalocyanines as molecular semiconductor thin films. Phys. Status Solidi (a) 2008, 205, 409–420. [Google Scholar] [CrossRef]
- Lu, C.-K.; Meng, H.-F. Hole doping by molecular oxygen in organic semiconductors: Band-structure calculations. Phys. Rev. B 2007, 75, 235206. [Google Scholar] [CrossRef] [Green Version]
- Nayak, P.K.; Rosenberg, R.; Barnea-Nehoshtan, L.; Cahen, D. O2 and organic semiconductors: Electronic effects. Org. Electron. 2013, 14, 966–972. [Google Scholar] [CrossRef]
- Bouvet, M.; Parra, V.; Suisse, J.-M. Molecular semiconductor-doped insulator (MSDI) heterojunctions as new transducers for chemical sensors. Eur. Phys. J. Appl. Phys. 2011, 56, 34103. [Google Scholar] [CrossRef]
- Ouedraogo, S.; Meunier-Prest, R.; Kumar, A.; Bayo-Bangoura, M.; Bouvet, M. Modulating the Electrical Properties of Organic Heterojunction Devices Based On Phthalocyanines for Ambipolar Sensors. ACS Sens. 2020, 5, 1849–1857. [Google Scholar] [CrossRef]
- Murdey, R.; Bouvet, M.; Sumimoto, M.; Sakaki, S.; Sato, N. Direct observation of the energy gap in lutetium bisphthalocyanine thin films. Synth. Met. 2009, 159, 1677–1681. [Google Scholar] [CrossRef]
- Chen, W.; Qi, D.-C.; Huang, H.; Gao, X.-Y.; Wee, A.T.S. Organic-Organic Heterojunction Interfaces: Effect of Molecular Orientation. Adv. Funct. Mater. 2010, 21, 410–424. [Google Scholar] [CrossRef]
- Lau, K.M.; Tang, J.X.; Sun, H.Y.; Lee, C.-S.; Lee, S.T.; Yan, D. Interfacial electronic structure of copper phthalocyanine and copper hexadecafluorophthalocyanine studied by photoemission. Appl. Phys. Lett. 2006, 88, 173513. [Google Scholar] [CrossRef]
- Wang, J.; Wang, H.; Yan, X.; Huang, H.; Yan, D. Organic heterojunction and its application for double channel field-effect transistors. Appl. Phys. Lett. 2005, 87, 93507. [Google Scholar] [CrossRef]
- Wang, H.; Wang, J.; Huang, H.; Yan, X.; Yan, D. Organic heterojunction with reverse rectifying characteristics and its application in field-effect transistors. Org. Electron. 2006, 7, 369–374. [Google Scholar] [CrossRef]
- Lee, Y.H.; Jang, M.; Lee, M.Y.; Kweon, O.Y.; Oh, J.H. Flexible Field-Effect Transistor-Type Sensors Based on Conjugated Molecules. Chemistry 2017, 3, 724–763. [Google Scholar] [CrossRef] [Green Version]
- Zhang, S.; Zhao, Y.; Du, X.; Chu, Y.; Zhang, S.; Huang, J. Gas Sensors Based on Nano/Microstructured Organic Field-Effect Transistors. Small 2019, 15, e1805196. [Google Scholar] [CrossRef]
- Wei, Z.; Xu, W.; Hu, W.; Zhu, D. Air-stable ambipolar organic field-effect transistor based on a novel bi-channel structure. J. Mater. Chem. 2008, 18, 2420. [Google Scholar] [CrossRef]
- Gaudillat, P.; Suisse, J.-M.; Bouvet, M. Humidity Insensitive Conductometric Sensors for Ammonia Sensing. Key Eng. Mater. 2014, 605, 181–184. [Google Scholar] [CrossRef]
- Mateos, M.; Meunier-Prest, R.; Heintz, O.; Herbst, F.; Suisse, J.-M.; Bouvet, M. Comprehensive Study of Poly(2,3,5,6-tetrafluoroaniline): From Electrosynthesis to Heterojunctions and Ammonia Sensing. ACS Appl. Mater. Interfaces 2018, 10, 19974–19986. [Google Scholar] [CrossRef]
- Huang, W.; Wang, Z.; Zhu, X.; Chi, L. Electrical gas sensors based on structured organic ultra-thin films and nanocrystals on solid state substrates. Nanoscale Horiz. 2016, 1, 383–393. [Google Scholar] [CrossRef]
- Lin, P.; Yan, F. Organic Thin-Film Transistors for Chemical and Biological Sensing. Adv. Mater. 2011, 24, 34–51. [Google Scholar] [CrossRef]
- Zhang, B.; Tai, H.L.; Xie, G.Z.; Li, X.; Zhang, H.N. The Investigation of a New NO2 OTFT Sensor Based on Heterojunction F16CuPc/CuPc Thin Films. Adv. Mater. Res. 2013, 721, 159–163. [Google Scholar] [CrossRef]
- Han, S.; Cheng, J.; Fan, H.; Yu, J.; Li, L. Achievement of High-Response Organic Field-Effect Transistor NO2 Sensor by Using the Synergistic Effect of ZnO/PMMA Hybrid Dielectric and CuPc/Pentacene Heterojunction. Sensors 2016, 16, 1763. [Google Scholar] [CrossRef] [PubMed] [Green Version]
- Fan, H.; Shi, W.; Yu, X.; Yu, J. High performance nitrogen dioxide sensor based on organic field-effect transistor utilizing ultrathin CuPc/PTCDI-C8 heterojunction. Synth. Met. 2016, 211, 161–166. [Google Scholar] [CrossRef]
- Huang, W.; Besar, K.; LeCover, R.; Rule, A.M.; Breysse, P.N.; Katz, H.E. Highly sensitive NH3 detection based on organic field-effect transistors with tris(pentafluorophenyl)borane as receptor. J. Am. Chem. Soc. 2012, 134, 14650–14653. [Google Scholar] [CrossRef] [PubMed] [Green Version]
- Wang, X.; Ji, S.; Wang, H.; Yan, D. Highly sensitive gas sensor enhanced by tuning the surface potential. Org. Electron. 2011, 12, 2230–2235. [Google Scholar] [CrossRef]
- Schuler, M.; Sauerwald, T.; Schütze, A.; Gaudillat, P.; Suisse, J.-M.; Bouvet, M. Selective Quantification of Humidity and Ammonia by Optical Excitation of Molecular Semiconductor-Doped Insulator (MSDI) Sensors. In Proceedings of the 2015 IEEE SENSORS, Busan, Korea, 1–4 November 2015; Institute of Electrical and Electronics Engineers (IEEE): Piscataway, NJ, USA, 2016; pp. 1–4. [Google Scholar]
- Şahin, Z.; Meunier-Prest, R.; Dumoulin, F.; Işci, Ü.; Bouvet, M. Alkylthio-tetrasubstituted μ-Nitrido Diiron Phthalocyanines: Spectroelectrochemistry, Electrical Properties, and Heterojunctions for Ammonia Sensing. Inorg. Chem. 2020, 59, 1057–1067. [Google Scholar] [CrossRef]
- Chen, Y.; Bouvet, M.; Sizun, T.; Barochi, G.; Rossignol, J.; Lesniewska, E. Enhanced chemosensing of ammonia based on the novel molecular semiconductor-doped insulator (MSDI) heterojunctions. Sens. Actuators B Chem. 2011, 155, 165–173. [Google Scholar] [CrossRef]
- Wannebroucq, A.; Ouedraogo, S.; Meunier-Prest, R.; Suisse, J.-M.; Bayo, M.; Bouvet, M. On the interest of ambipolar materials for gas sensing. Sens. Actuators B Chem. 2018, 258, 657–664. [Google Scholar] [CrossRef] [Green Version]
- Wannebroucq, A.; Meunier-Prest, R.; Chambron, J.-C.; Brachais, C.-H.; Suisse, J.-M.; Bouvet, M. Synthesis and characterization of fluorophthalocyanines bearing four 2-(2-thienyl)ethoxy moieties: From the optimization of the fluorine substitution to chemosensing. RSC Adv. 2017, 7, 41272–41281. [Google Scholar] [CrossRef] [Green Version]
- Bouvet, M.; Xiong, H.; Parra, V. Molecular semiconductor-doped insulator (MSDI) heterojunctions: Oligothiophene/bisphtalocyanine (LuPc2) and perylene/bisphthalocyanine as new structures for gas sensing. Sens. Actuators B Chem. 2010, 145, 501–506. [Google Scholar] [CrossRef]
- Gaudillat, P.; Wannebroucq, A.; Suisse, J.-M.; Bouvet, M. Bias and humidity effects on the ammonia sensing of perylene derivative/lutetium bisphthalocyanine MSDI heterojunctions. Sens. Actuators B Chem. 2016, 222, 910–917. [Google Scholar] [CrossRef]
- Wannebroucq, A.; Gruntz, G.; Suisse, J.-M.; Nicolas, Y.; Meunier-Prest, R.; Mateos, M.; Toupance, T.; Bouvet, M. New n-type molecular semiconductor–doped insulator (MSDI) heterojunctions combining a triphenodioxazine (TPDO) and the lutetium bisphthalocyanine (LuPc2) for ammonia sensing. Sens. Actuators B 2018, 255, 1694–1700. [Google Scholar] [CrossRef] [Green Version]
- Kwon, H.; Yoo, H.; Nakano, M.; Takimiya, K.; Kim, J.-J.; Kim, J.K. Gate-tunable gas sensing behaviors in air-stable ambipolar organic thin-film transistors. RSC Adv. 2020, 10, 1910–1916. [Google Scholar] [CrossRef] [Green Version]
- Guo, Z.; Wang, B.; Wang, X.; Li, Y.; Gai, S.; Wu, Y.; Cheng, X. A high-sensitive room temperature gas sensor based on cobalt phthalocyanines and reduced graphene oxide nanohybrids for the ppb-levels of ammonia detection. RSC Adv. 2019, 9, 37518–37525. [Google Scholar] [CrossRef]
- Zhou, X.; Wang, X.; Wang, B.; Chen, Z.; He, C.; Wu, Y. Preparation, characterization and NH3-sensing properties of reduced graphene oxide/copper phthalocyanine hybrid material. Sens. Actuators B Chem. 2014, 193, 340–348. [Google Scholar] [CrossRef]
- Peng, R.; Li, Y.; Liu, T.; Sun, Q.; Si, P.; Zhang, L.; Ci, L. Reduced graphene oxide/SnO2@Au heterostructure for enhanced ammonia gas sensing. Chem. Phys. Lett. 2019, 737, 136829. [Google Scholar] [CrossRef]
Devices | RR [%] | Gas Concentration [ppm] | S [% ppm−1] | LOD [ppm] | Range [ppm] | Ref. |
---|---|---|---|---|---|---|
Cu(F16Pc)/CuPc OFET | 18 | 20 (NO2) | [66] | |||
Pentacene/CuPc OFET | 193 | 15 (NO2) | 0.5–15 | [67] | ||
CuPc/PTCDI-C8 OFET | 45 | 2 (NO2) | 2–30 | [68] | ||
CuPc/TPFB OFET | 33 | 4.5 (NH3) | 0.35 | 0.45–20 | [69] | |
CoPc/TPFB OFET | 37 | 4.5 (NH3) | 0.35 | 0.45–20 | [69] | |
p-6P/PTCDI-ph/VOPc OFET | 90 | 5 (NO2) | 5–30 | [44] | ||
CuPc(COOC8H17)8/CuPc(OC8H17)8 OFET | 300 | 600 (ethanol) | 0.49 | 100 | 200–1400 | [70] |
TiOPc/Cu(F16Pc) OFET | 35 | 4 (NO2) | 0.25 | 1–5 | [17] | |
Co(Cl8Pc)/LuPc2 MSDI | 58 | 90 (NH3) | 1.48 | 0.25 | 1–9 | [53] |
Cu(Cl8Pc)/LuPc2 MSDI | 35 | 90 (NH3) | 30–90 | [53] | ||
Cu(Cl8Pc)/LuPc2 MSDI | 77 | 30 (NH3) | 0–30 | [53] | ||
N-(ttbFePc)2/LuPc2 MSDI | 11 | 30 (NH3) | 10–90 | [72] | ||
TFBz/Cu(Cl8Pc)/LuPc2 MSDI | 55 | 90 (NH3) | 0.14 | 1.2 | 1–9 | [14] |
Bz/Cu(F16Pc)/LuPc2 MSDI | 67 | 90 (NH3) | 1.5 | 0.28 | 1–9 | [14] |
DMBz/Cu(F16Pc)/LuPc2 MSDI | 138 | 90 (NH3) | 3 | 0.14 | 1–9 | [14] |
TFEBz/Cu(F16Pc)/LuPc2 MSDI | 50 | 90 (NH3) | 1.1 | 2 | 1–9 | [14] |
CuPc/Eu2[Pc(15C5)4]2[Pc(OC10H21)8] MSDI | 5 | 50 (NH3) | 15–800 | [73] | ||
PTCDA/LuPc2 MSDI | 34 | 90 (NH3) | 0.6 | 10–30 | [77] | |
TPDO/LuPc2 MSDI | 26 | 90 (NH3) | 0.2 | 30–90 | [78] | |
PTCDI/ LuPc2 MSDI | 10 | 0.4 (ozone) | [76] | |||
PTCDI/ LuPc2 MSDI | 20 | 100 (NH3) | 100–800 | [76] | ||
PTFA/LuPc2 MSDI | 14 | 90 (NH3) | 1.05 | 0.45 | 1–6 | [63] |
PDMA/LuPc2 MSDI | 14 | 90 (NH3) | 2.23 | 0.314 | 1–6 | [15] |
© 2020 by the authors. Licensee MDPI, Basel, Switzerland. This article is an open access article distributed under the terms and conditions of the Creative Commons Attribution (CC BY) license (http://creativecommons.org/licenses/by/4.0/).
Share and Cite
Kumar, A.; Meunier-Prest, R.; Bouvet, M. Organic Heterojunction Devices Based on Phthalocyanines: A New Approach to Gas Chemosensing. Sensors 2020, 20, 4700. https://doi.org/10.3390/s20174700
Kumar A, Meunier-Prest R, Bouvet M. Organic Heterojunction Devices Based on Phthalocyanines: A New Approach to Gas Chemosensing. Sensors. 2020; 20(17):4700. https://doi.org/10.3390/s20174700
Chicago/Turabian StyleKumar, Abhishek, Rita Meunier-Prest, and Marcel Bouvet. 2020. "Organic Heterojunction Devices Based on Phthalocyanines: A New Approach to Gas Chemosensing" Sensors 20, no. 17: 4700. https://doi.org/10.3390/s20174700
APA StyleKumar, A., Meunier-Prest, R., & Bouvet, M. (2020). Organic Heterojunction Devices Based on Phthalocyanines: A New Approach to Gas Chemosensing. Sensors, 20(17), 4700. https://doi.org/10.3390/s20174700