Proteomics in Inherited Metabolic Disorders
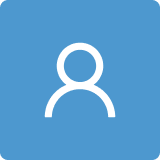
Round 1
Reviewer 1 Report
In this review article, the authors discussed how proteomics could contribute to the study of inherited metabolic disorders (IMDs). The authors summarized the current approaches in qualitative and quantitative proteomics, identified the limitations of each method, and highlighted some of the most important discoveries in the field of IMDs obtained using proteomics approaches. I believe this manuscript would be a valuable resource for audience interested in applying proteomics to study the pathophysiology of IMDs.
Minor issues:
1. Spacing between words is missing in many places. This likely can be addressed during proofreading.
2. The charge states of peaks in the mass spectrum in Figure 2 seem unnecessary and confusing especially when they are increasing from +2 to +16 over the m/z axis.
3. As most of the disease-related proteins in IMDs are metabolic enzymes, it would be natural to include some discussions regarding the approaches and recent discoveries made by activity-based probes and activity-based proteomics approaches.
Author Response
In this review article, the authors discussed how proteomics could contribute to the study of inherited metabolic disorders (IMDs). The authors summarized the current approaches in qualitative and quantitative proteomics, identified the limitations of each method, and highlighted some of the most important discoveries in the field of IMDs obtained using proteomics approaches. I believe this manuscript would be a valuable resource for audience interested in applying proteomics to study the pathophysiology of IMDs.
Minor issues:
- Spacing between words is missing in many places. This likely can be addressed during proofreading.
We appreciate the reviewer’s comment. This error has been rectified.
- The charge states of peaks in the mass spectrum in Figure 2 seem unnecessary and confusing especially when they are increasing from +2 to +16 over the m/z axis.
We agree with the reviewer’s comment and have removed the charges in the figure.
- As most of the disease-related proteins in IMDs are metabolic enzymes, it would be natural to include some discussions regarding the approaches and recent discoveries made by activity-based probes and activity-based proteomics approaches.
We agree with this comment and have included a new subsection in section 2.1 Qualitative and Quantitative Proteomics
2.1.4 Affinity-based (probes and antibodies) proteomics approaches [35–38]
Affinity-based proteomics is a relatively new field of proteomics that seeks to characterize protein activity and protein-protein interaction. This method also allows monitoring of the functional regulation of enzymes in complex proteomes. This technology utilizes small molecule activity-based probes (APBs) or protein antibodies. The probe molecules covalently modify the enzyme active site and allow detection and affinity purification of a target enzyme population. Affinity-based antibodies are mainly generated using microarrays or directly by antibody immunoprecipitation of the whole sample. After affinity purification (pulldown based on probes or antibodies), substrates and their exact cleavage sites are identified by mass spectrometry. Moreover, cleavage sites can be quantitatively evaluated by incorporation of quantitative proteomic techniques such as iTRAQ and SILAC [39]. Thus, this technology is highly selective and can be used for analysis of complex samples such as biological fluids, cell lysates, intact cells, and even whole organisms, and can therefore be applied to the study of IMDs.
All activity-based probes share a similar structure[35,39]. They have elements required for targeting, modification, and detection of labeled proteins. Structurally, those elements can be divided into the reactive group, linker, and tag. The reactive functional group provides a covalent attachment to the catalytic residue of the enzyme active site. The linker function separates the reactive functional group from the tag. Finally, the tag enables visualization and/or purification of labeled proteins.
For the antibody microarray, the data set correlates directly with the number of antibodies included on the array and the range of their specificities [37].
Technologies that reveal the most abundant proteins found in certain samples types (e.g. serum) are crucial for the development of biomarkers that can be used as measurable indicators of disease severity and progression, patient stratification, and drug development. Proximity Extension Assay (PEA) is a novel approach based in the interaction between protein antibodies and DNA [38] that has been commercially developed for the analysis of secreted proteins in serum and blood plasma. This technology can translate protein information into actionable knowledge by linking protein-specific antibodies to DNA-encoded tags. It offers high specificity and sensitivity (sub-pg/ml), enabling high multiplex assays with coverage across a broad dynamic range (~9 log), while consuming minimal quantities of sample. In PEA, matched pairs of oligonucleotide-labeled antibodies bind to their target antigens in a pairwise manner. Upon antibody binding, the matched oligonucleotides are brought into proximity, and using a DNA polymerase a PCR target sequence is created, amplified, detected, and quantified. This downstream process analysis is usually carried out by qPCR or by techniques such as next-generation sequencing (NGS).
The above are all recent technologies, and the coming years will likely see continued growth in the development and application of affinity-based proteomic approaches. The use of probes that target diverse enzyme families has led to a range of applications for activity-based proteomics, in the same way that linking proteins to proteins has provided information about the interactome and protein-DNA interactions have provided further knowledge about cell processes. Therefore, as in other proteomics approaches, comparison of enzyme activity profiles and protein-protein or protein-DNA interactions (based on antibody precipitation) in healthy versus diseased samples can aid the identification of novel biomarkers and drug targets. The results obtained to date show that affinity-based proteomics offer great promise as imaging tools for disease diagnosis and preclinical and clinical testing of therapeutic agents in vivo.
We have also included a new study in table 1 in which the authors used affinity-based methods applied to IMDs, in this case Duchenne muscular dystrophy.
Duchenne muscular dystrophy (DMD; OMIM310200) is a rare X-linked genetic disease with an incidence rate of 1:5,000. DMD is caused by frame-disrupting mutations in the gene encoding dystrophin, resulting in loss of dystrophin [90]. Affected boys typically present clinical signs in the first few years of life, with features suggestive of muscle weakness and often with global developmental delay. Progressive muscle weakness leads to loss of ambulation by the age of 10, and if untreated, to fatal cardio respiratory insufficiency by late adolescence. Establishing a correct diagnosis in dystrophinopathies requires a multidisciplinary approach involving pediatricians, geneticists, and neurologists to define the severity of the clinical phenotype by means of genetic, enzymatic, and immunohistochemical tests [90]. The application of proteomics to the study of dystrophinopathies could facilitate both diagnosis and treatment. In 2014 Ayoglu et al. [36] explored the possibility of identifying circulating candidate protein markers in rare diseases applying an affinity-based proteomics approach to generate a proteomic profile in blood samples from muscular dystrophy patients versus controls. The authors used an antibody bead array platform with 384 antibodies. Based on the results obtained from analysis of both serum and plasma, they identified 11 proteins associated with muscular dystrophy, of which 4 were upregulated in blood from muscular dystrophy patients: carbonic anhydrase III (CA3), myosin light chain 3 (MYL3), mitochondrial malate dehydrogenase 2 (MDH2), and electron transfer flavoprotein A (ETFA). These interesting data could aid the development of new clinical tools for management of dystrophinopathies.
Author Response File: Author Response.pdf
Reviewer 2 Report
In the review "Proteomics in Inherited Metabolic Disorders" Maria del Pilar Chantada-Vázquez and collegues describe the application of proteomics methods to the study of Inherited Metabolic Disorders (IMD).
The manuscript is well written and comprehensive, however my suggestion is to either change the title to "Mass Spectrometry (MS) in IMD" or to include in the discussion studies based on Affinity Proteomics as well as MS.
The general description about proteomics methods could be revised cutting (or only mentioning) 2D-PAGE which is a relatively old technique, while including methods such as high multiplexed protein profiling using Proximity Extension Assays (PEA) or Somascan.
A few example of possibly relevant studies are:
1. Cells. 2022 Mar 7;11(5):913. doi: 10.3390/cells11050913.
Hongge Wang et al. Evaluation of Neurofilament Light Chain as a Biomarker of Neurodegeneration in X-Linked Childhood Cerebral Adrenoleukodystrophy
2. Blood Adv. 2021 Aug 24;5(16):3092-3101. doi:10.1182/bloodadvances.2020003824.
Brigitte T.A.Long-term effect of hematopoietic cell transplantation on systemic inflammation in patients with mucopolysaccharidoses
Minor Comments: Figure 1. The sample type should be above the two blocks "Bottom-up" and "Top down" because in the current way it is confusing. Figure 4. I would specify the difference between untargeted DIA (eg SWATH) and targeted DIA (SRM/MRM and PRM). Figure 3 and Figure 4. When talking about targeted MS or quantitative MS the authors should mention that methods such as SRM/MRM and PRM are not simply label free, but they can be quantitative because they imply the use of an internal standard (labeled peptide), while methods based on isobaric or chemical labeling remain semiquantitative.
Author Response
In the review "Proteomics in Inherited Metabolic Disorders" Maria del Pilar Chantada-Vázquez and collegues describe the application of proteomics methods to the study of Inherited Metabolic Disorders (IMD).
The manuscript is well written and comprehensive, however my suggestion is to either change the title to "Mass Spectrometry (MS) in IMD" or to include in the discussion studies based on Affinity Proteomics as well as MS.
We appreciate the comment. We agree with the reviewer and have included a new subsection in section 2.1 Qualitative and Quantitative Proteomics
2.1.4 Affinity-based (probes and antibodies) proteomics approaches [35–38]
Affinity-based proteomics is a relatively new field of proteomics that seeks to characterize protein activity and protein-protein interaction. This method also allows monitoring of the functional regulation of enzymes in complex proteomes. This technology utilizes small molecule activity-based probes (APBs) or protein antibodies. The probe molecules covalently modify the enzyme active site and allow detection and affinity purification of a target enzyme population. Affinity-based antibodies are mainly generated using microarrays or directly by antibody immunoprecipitation of the whole sample. After affinity purification (pulldown based on probes or antibodies), substrates and their exact cleavage sites are identified by mass spectrometry. Moreover, cleavage sites can be quantitatively evaluated by incorporation of quantitative proteomic techniques such as iTRAQ and SILAC [39]. Thus, this technology is highly selective and can be used for analysis of complex samples such as biological fluids, cell lysates, intact cells, and even whole organisms, and can therefore be applied to the study of IMDs.
All activity-based probes share a similar structure[35,39]. They have elements required for targeting, modification, and detection of labeled proteins. Structurally, those elements can be divided into the reactive group, linker, and tag. The reactive functional group provides a covalent attachment to the catalytic residue of the enzyme active site. The linker function separates the reactive functional group from the tag. Finally, the tag enables visualization and/or purification of labeled proteins.
For the antibody microarray, the data set correlates directly with the number of antibodies included on the array and the range of their specificities [37].
Technologies that reveal the most abundant proteins found in certain samples types (e.g. serum) are crucial for the development of biomarkers that can be used as measurable indicators of disease severity and progression, patient stratification, and drug development. Proximity Extension Assay (PEA) is a novel approach based in the interaction between protein antibodies and DNA [38] that has been commercially developed for the analysis of secreted proteins in serum and blood plasma. This technology can translate protein information into actionable knowledge by linking protein-specific antibodies to DNA-encoded tags. It offers high specificity and sensitivity (sub-pg/ml), enabling high multiplex assays with coverage across a broad dynamic range (~9 log), while consuming minimal quantities of sample. In PEA, matched pairs of oligonucleotide-labeled antibodies bind to their target antigens in a pairwise manner. Upon antibody binding, the matched oligonucleotides are brought into proximity, and using a DNA polymerase a PCR target sequence is created, amplified, detected, and quantified. This downstream process analysis is usually carried out by qPCR or by techniques such as next-generation sequencing (NGS).
The above are all recent technologies, and the coming years will likely see continued growth in the development and application of affinity-based proteomic approaches. The use of probes that target diverse enzyme families has led to a range of applications for activity-based proteomics, in the same way that linking proteins to proteins has provided information about the interactome and protein-DNA interactions have provided further knowledge about cell processes. Therefore, as in other proteomics approaches, comparison of enzyme activity profiles and protein-protein or protein-DNA interactions (based on antibody precipitation) in healthy versus diseased samples can aid the identification of novel biomarkers and drug targets. The results obtained to date show that affinity-based proteomics offer great promise as imaging tools for disease diagnosis and preclinical and clinical testing of therapeutic agents in vivo.
We have also included a new study in table 1 in which the authors used affinity-based methods applied to IMDs, in this case Duchenne muscular dystrophy.
Duchenne muscular dystrophy (DMD; OMIM310200) is a rare X-linked genetic disease with an incidence rate of 1:5,000. DMD is caused by frame-disrupting mutations in the gene encoding dystrophin, resulting in loss of dystrophin [90]. Affected boys typically present clinical signs in the first few years of life, with features suggestive of muscle weakness and often with global developmental delay. Progressive muscle weakness leads to loss of ambulation by the age of 10, and if untreated, to fatal cardio respiratory insufficiency by late adolescence. Establishing a correct diagnosis in dystrophinopathies requires a multidisciplinary approach involving pediatricians, geneticists, and neurologists to define the severity of the clinical phenotype by means of genetic, enzymatic, and immunohistochemical tests [90]. The application of proteomics to the study of dystrophinopathies could facilitate both diagnosis and treatment. In 2014 Ayoglu et al. [36] explored the possibility of identifying circulating candidate protein markers in rare diseases applying an affinity-based proteomics approach to generate a proteomic profile in blood samples from muscular dystrophy patients versus controls. The authors used an antibody bead array platform with 384 antibodies. Based on the results obtained from analysis of both serum and plasma, they identified 11 proteins associated with muscular dystrophy, of which 4 were upregulated in blood from muscular dystrophy patients: carbonic anhydrase III (CA3), myosin light chain 3 (MYL3), mitochondrial malate dehydrogenase 2 (MDH2), and electron transfer flavoprotein A (ETFA). These interesting data could aid the development of new clinical tools for management of dystrophinopathies.
The general description about proteomics methods could be revised cutting (or only mentioning) 2D-PAGE which is a relatively old technique, while including methods such as high multiplexed protein profiling using Proximity Extension Assays (PEA) or Somascan.
A few examples of possibly relevant studies are:
- Cells. 2022 Mar 7;11(5):913. doi: 10.3390/cells11050913.
Hongge Wang et al. Evaluation of Neurofilament Light Chain as a Biomarker of Neurodegeneration in X-Linked Childhood Cerebral Adrenoleukodystrophy
- Blood Adv. 2021 Aug 24;5(16):3092-3101. doi:10.1182/bloodadvances.2020003824.
Brigitte T.A.Long-term effect of hematopoietic cell transplantation on systemic inflammation in patients with mucopolysaccharidoses
We appreciate the reviewer’s comments. We have removed the 2d-PAGE paragraph and included discussion of the PEA technique in section 2.1.4, as referred to in the previous response. The two examples of this technique applied to the study of IMDs were included in table 1 and discussed in the text as we indicated below.
In 2020 van den Broek et al.[71] investigated systemic inflammation in untreated MPS I patients and evaluated the effect of HCT (hematopoietic cell transplantation) on systemic inflammation. The authors used dried blood spot samples from patients with HCT-treated MPS using a OLINK Proseek Multiplex inflammation platform. In total, 92 markers of the OLINK inflammation panel were measured in MPS patients compared with those of age-matched control subjects. The authors observed normal leukocyte enzyme activity levels in 93% of patients post-HCT. Moreover, pretransplant samples showed clear differences between patients and controls. The protein markers that distinguished control subjects from patients pre-HCT were mainly pro-inflammatory (50%) or related to bone homeostasis and extracellular matrix degradation (33%). After 10 years of follow-up, only 5 markers (receptor activator of nuclear factor kappa-B ligand, osteoprotegerin, axis inhibition protein 1 [AXIN1], stem cell factor, and Fms-related tyrosine kinase 3 ligand) remained significantly increased, with a large fold-change difference between MPS I patients and control subjects.
In 2022 Wang et al.[79] used the OLINK Proximity Extension Assay to analyze the cerebral spinal fluid (CSF) of young males with CALD, and performed a comparative analysis using plasma samples. Using the Target 96 Neuro Exploratory panel they found that levels of 5 proteins were significantly increased in CSF. Only for neurofilament light chain (NfL) was a significant correlation observed between CSF and plasma samples. Young males with CALD showed a 11.3-fold increase in plasma NfL compared with controls. Moreover, 9 of 11 young males with CALD who underwent HCT showed a decrease in plasma NfL levels after 1 year of treatment compared with pre-HCT levels. The authors concluded that plasma NfL could constitute a useful determinant of outcomes in CALD
Minor Comments: Figure 1. The sample type should be above the two blocks "Bottom-up" and "Top down" because in the current way it is confusing.
We agree with the reviewer and have edited the figures accordingly.
Figure 4. I would specify the difference between untargeted DIA (eg SWATH) and targeted DIA (SRM/MRM and PRM).
We agree with the reviewer, we change figure 4 adding untargeted DIA for SWATH and targeted DIA for SRM/MRM and PRM. Moreover, the following text has been added to the figure legend:
Figure 4: DDA and DIA mode in proteomic analysis
In DDA mode, extracted proteins are digested and directly analyzed by single-shot DDA. The acquired data is searched against a database of known protein sequences and further processed using diverse software tools. In DIA mode two distinct forms of proteomic analysis can be performed: SWATH-MS (untargeted DIA) and SRM/MRM (targeted DIA). In SWATH-MS extracted proteins are digested and directly analyzed by single-shot analysis in order to generate the spectral library. Once the library is generated a multi-window running method is applied to individual samples: wide isolation windows span the entire MS1 m/z range and all precursor ions in the library that are found in each isolation window are fragmented in MS2. All proteins found in the library are quantified (untargeted) and all samples are processed using data analysis software to obtain the quantitative data. In MRM and SRM, the precursor ions to be analyzed are fixed (targeted) by the user, and only these are fragmented in MS2.
Figure 3 and Figure 4. When talking about targeted MS or quantitative MS the authors should mention that methods such as SRM/MRM and PRM are not simply label free, but they can be quantitative because they imply the use of an internal standard (labeled peptide), while methods based on isobaric or chemical labeling remain semiquantitative.
We agree with the reviewer: it is prudent to emphasize this important difference. The following text has been included at the end of point 2.1.3:
It should be noted that methods such as MRM/SRM/PRM-MS allow for quantification of absolute protein levels. Other previously described methods such as label-based (e.g. ITRAQ, SILAC) and non-label-based methods (e.g. SWATH) are semi quantitative methods that only indicate the fold change in protein levels between two conditions.
Targeted MS/MS allows absolute quantification of targeted proteins using isotopically labelled peptide standards (AQUA) [30–32] or protein standard absolute quantification (PSAQ) [31,33,34]. Using isotope standards simplifies sample preparation and minimizes variation between mass spectrometry devices, thereby increasing the reliability and accuracy of results.
In the review "Proteomics in Inherited Metabolic Disorders" Maria del Pilar Chantada-Vázquez and collegues describe the application of proteomics methods to the study of Inherited Metabolic Disorders (IMD).
The manuscript is well written and comprehensive, however my suggestion is to either change the title to "Mass Spectrometry (MS) in IMD" or to include in the discussion studies based on Affinity Proteomics as well as MS.
We appreciate the comment. We agree with the reviewer and have included a new subsection in section 2.1 Qualitative and Quantitative Proteomics
2.1.4 Affinity-based (probes and antibodies) proteomics approaches [35–38]
Affinity-based proteomics is a relatively new field of proteomics that seeks to characterize protein activity and protein-protein interaction. This method also allows monitoring of the functional regulation of enzymes in complex proteomes. This technology utilizes small molecule activity-based probes (APBs) or protein antibodies. The probe molecules covalently modify the enzyme active site and allow detection and affinity purification of a target enzyme population. Affinity-based antibodies are mainly generated using microarrays or directly by antibody immunoprecipitation of the whole sample. After affinity purification (pulldown based on probes or antibodies), substrates and their exact cleavage sites are identified by mass spectrometry. Moreover, cleavage sites can be quantitatively evaluated by incorporation of quantitative proteomic techniques such as iTRAQ and SILAC [39]. Thus, this technology is highly selective and can be used for analysis of complex samples such as biological fluids, cell lysates, intact cells, and even whole organisms, and can therefore be applied to the study of IMDs.
All activity-based probes share a similar structure[35,39]. They have elements required for targeting, modification, and detection of labeled proteins. Structurally, those elements can be divided into the reactive group, linker, and tag. The reactive functional group provides a covalent attachment to the catalytic residue of the enzyme active site. The linker function separates the reactive functional group from the tag. Finally, the tag enables visualization and/or purification of labeled proteins.
For the antibody microarray, the data set correlates directly with the number of antibodies included on the array and the range of their specificities [37].
Technologies that reveal the most abundant proteins found in certain samples types (e.g. serum) are crucial for the development of biomarkers that can be used as measurable indicators of disease severity and progression, patient stratification, and drug development. Proximity Extension Assay (PEA) is a novel approach based in the interaction between protein antibodies and DNA [38] that has been commercially developed for the analysis of secreted proteins in serum and blood plasma. This technology can translate protein information into actionable knowledge by linking protein-specific antibodies to DNA-encoded tags. It offers high specificity and sensitivity (sub-pg/ml), enabling high multiplex assays with coverage across a broad dynamic range (~9 log), while consuming minimal quantities of sample. In PEA, matched pairs of oligonucleotide-labeled antibodies bind to their target antigens in a pairwise manner. Upon antibody binding, the matched oligonucleotides are brought into proximity, and using a DNA polymerase a PCR target sequence is created, amplified, detected, and quantified. This downstream process analysis is usually carried out by qPCR or by techniques such as next-generation sequencing (NGS).
The above are all recent technologies, and the coming years will likely see continued growth in the development and application of affinity-based proteomic approaches. The use of probes that target diverse enzyme families has led to a range of applications for activity-based proteomics, in the same way that linking proteins to proteins has provided information about the interactome and protein-DNA interactions have provided further knowledge about cell processes. Therefore, as in other proteomics approaches, comparison of enzyme activity profiles and protein-protein or protein-DNA interactions (based on antibody precipitation) in healthy versus diseased samples can aid the identification of novel biomarkers and drug targets. The results obtained to date show that affinity-based proteomics offer great promise as imaging tools for disease diagnosis and preclinical and clinical testing of therapeutic agents in vivo.
We have also included a new study in table 1 in which the authors used affinity-based methods applied to IMDs, in this case Duchenne muscular dystrophy.
Duchenne muscular dystrophy (DMD; OMIM310200) is a rare X-linked genetic disease with an incidence rate of 1:5,000. DMD is caused by frame-disrupting mutations in the gene encoding dystrophin, resulting in loss of dystrophin [90]. Affected boys typically present clinical signs in the first few years of life, with features suggestive of muscle weakness and often with global developmental delay. Progressive muscle weakness leads to loss of ambulation by the age of 10, and if untreated, to fatal cardio respiratory insufficiency by late adolescence. Establishing a correct diagnosis in dystrophinopathies requires a multidisciplinary approach involving pediatricians, geneticists, and neurologists to define the severity of the clinical phenotype by means of genetic, enzymatic, and immunohistochemical tests [90]. The application of proteomics to the study of dystrophinopathies could facilitate both diagnosis and treatment. In 2014 Ayoglu et al. [36] explored the possibility of identifying circulating candidate protein markers in rare diseases applying an affinity-based proteomics approach to generate a proteomic profile in blood samples from muscular dystrophy patients versus controls. The authors used an antibody bead array platform with 384 antibodies. Based on the results obtained from analysis of both serum and plasma, they identified 11 proteins associated with muscular dystrophy, of which 4 were upregulated in blood from muscular dystrophy patients: carbonic anhydrase III (CA3), myosin light chain 3 (MYL3), mitochondrial malate dehydrogenase 2 (MDH2), and electron transfer flavoprotein A (ETFA). These interesting data could aid the development of new clinical tools for management of dystrophinopathies.
The general description about proteomics methods could be revised cutting (or only mentioning) 2D-PAGE which is a relatively old technique, while including methods such as high multiplexed protein profiling using Proximity Extension Assays (PEA) or Somascan.
A few examples of possibly relevant studies are:
- Cells. 2022 Mar 7;11(5):913. doi: 10.3390/cells11050913.
Hongge Wang et al. Evaluation of Neurofilament Light Chain as a Biomarker of Neurodegeneration in X-Linked Childhood Cerebral Adrenoleukodystrophy
- Blood Adv. 2021 Aug 24;5(16):3092-3101. doi:10.1182/bloodadvances.2020003824.
Brigitte T.A.Long-term effect of hematopoietic cell transplantation on systemic inflammation in patients with mucopolysaccharidoses
We appreciate the reviewer’s comments. We have removed the 2d-PAGE paragraph and included discussion of the PEA technique in section 2.1.4, as referred to in the previous response. The two examples of this technique applied to the study of IMDs were included in table 1 and discussed in the text as we indicated below.
In 2020 van den Broek et al.[71] investigated systemic inflammation in untreated MPS I patients and evaluated the effect of HCT (hematopoietic cell transplantation) on systemic inflammation. The authors used dried blood spot samples from patients with HCT-treated MPS using a OLINK Proseek Multiplex inflammation platform. In total, 92 markers of the OLINK inflammation panel were measured in MPS patients compared with those of age-matched control subjects. The authors observed normal leukocyte enzyme activity levels in 93% of patients post-HCT. Moreover, pretransplant samples showed clear differences between patients and controls. The protein markers that distinguished control subjects from patients pre-HCT were mainly pro-inflammatory (50%) or related to bone homeostasis and extracellular matrix degradation (33%). After 10 years of follow-up, only 5 markers (receptor activator of nuclear factor kappa-B ligand, osteoprotegerin, axis inhibition protein 1 [AXIN1], stem cell factor, and Fms-related tyrosine kinase 3 ligand) remained significantly increased, with a large fold-change difference between MPS I patients and control subjects.
In 2022 Wang et al.[79] used the OLINK Proximity Extension Assay to analyze the cerebral spinal fluid (CSF) of young males with CALD, and performed a comparative analysis using plasma samples. Using the Target 96 Neuro Exploratory panel they found that levels of 5 proteins were significantly increased in CSF. Only for neurofilament light chain (NfL) was a significant correlation observed between CSF and plasma samples. Young males with CALD showed a 11.3-fold increase in plasma NfL compared with controls. Moreover, 9 of 11 young males with CALD who underwent HCT showed a decrease in plasma NfL levels after 1 year of treatment compared with pre-HCT levels. The authors concluded that plasma NfL could constitute a useful determinant of outcomes in CALD
Minor Comments: Figure 1. The sample type should be above the two blocks "Bottom-up" and "Top down" because in the current way it is confusing.
We agree with the reviewer and have edited the figures accordingly.
Figure 4. I would specify the difference between untargeted DIA (eg SWATH) and targeted DIA (SRM/MRM and PRM).
We agree with the reviewer, we change figure 4 adding untargeted DIA for SWATH and targeted DIA for SRM/MRM and PRM. Moreover, the following text has been added to the figure legend:
Figure 4: DDA and DIA mode in proteomic analysis
In DDA mode, extracted proteins are digested and directly analyzed by single-shot DDA. The acquired data is searched against a database of known protein sequences and further processed using diverse software tools. In DIA mode two distinct forms of proteomic analysis can be performed: SWATH-MS (untargeted DIA) and SRM/MRM (targeted DIA). In SWATH-MS extracted proteins are digested and directly analyzed by single-shot analysis in order to generate the spectral library. Once the library is generated a multi-window running method is applied to individual samples: wide isolation windows span the entire MS1 m/z range and all precursor ions in the library that are found in each isolation window are fragmented in MS2. All proteins found in the library are quantified (untargeted) and all samples are processed using data analysis software to obtain the quantitative data. In MRM and SRM, the precursor ions to be analyzed are fixed (targeted) by the user, and only these are fragmented in MS2.
Figure 3 and Figure 4. When talking about targeted MS or quantitative MS the authors should mention that methods such as SRM/MRM and PRM are not simply label free, but they can be quantitative because they imply the use of an internal standard (labeled peptide), while methods based on isobaric or chemical labeling remain semiquantitative.
We agree with the reviewer: it is prudent to emphasize this important difference. The following text has been included at the end of point 2.1.3:
It should be noted that methods such as MRM/SRM/PRM-MS allow for quantification of absolute protein levels. Other previously described methods such as label-based (e.g. ITRAQ, SILAC) and non-label-based methods (e.g. SWATH) are semi quantitative methods that only indicate the fold change in protein levels between two conditions.
Targeted MS/MS allows absolute quantification of targeted proteins using isotopically labelled peptide standards (AQUA) [30–32] or protein standard absolute quantification (PSAQ) [31,33,34]. Using isotope standards simplifies sample preparation and minimizes variation between mass spectrometry devices, thereby increasing the reliability and accuracy of results.
In the review "Proteomics in Inherited Metabolic Disorders" Maria del Pilar Chantada-Vázquez and collegues describe the application of proteomics methods to the study of Inherited Metabolic Disorders (IMD).
The manuscript is well written and comprehensive, however my suggestion is to either change the title to "Mass Spectrometry (MS) in IMD" or to include in the discussion studies based on Affinity Proteomics as well as MS.
We appreciate the comment. We agree with the reviewer and have included a new subsection in section 2.1 Qualitative and Quantitative Proteomics
2.1.4 Affinity-based (probes and antibodies) proteomics approaches [35–38]
Affinity-based proteomics is a relatively new field of proteomics that seeks to characterize protein activity and protein-protein interaction. This method also allows monitoring of the functional regulation of enzymes in complex proteomes. This technology utilizes small molecule activity-based probes (APBs) or protein antibodies. The probe molecules covalently modify the enzyme active site and allow detection and affinity purification of a target enzyme population. Affinity-based antibodies are mainly generated using microarrays or directly by antibody immunoprecipitation of the whole sample. After affinity purification (pulldown based on probes or antibodies), substrates and their exact cleavage sites are identified by mass spectrometry. Moreover, cleavage sites can be quantitatively evaluated by incorporation of quantitative proteomic techniques such as iTRAQ and SILAC [39]. Thus, this technology is highly selective and can be used for analysis of complex samples such as biological fluids, cell lysates, intact cells, and even whole organisms, and can therefore be applied to the study of IMDs.
All activity-based probes share a similar structure[35,39]. They have elements required for targeting, modification, and detection of labeled proteins. Structurally, those elements can be divided into the reactive group, linker, and tag. The reactive functional group provides a covalent attachment to the catalytic residue of the enzyme active site. The linker function separates the reactive functional group from the tag. Finally, the tag enables visualization and/or purification of labeled proteins.
For the antibody microarray, the data set correlates directly with the number of antibodies included on the array and the range of their specificities [37].
Technologies that reveal the most abundant proteins found in certain samples types (e.g. serum) are crucial for the development of biomarkers that can be used as measurable indicators of disease severity and progression, patient stratification, and drug development. Proximity Extension Assay (PEA) is a novel approach based in the interaction between protein antibodies and DNA [38] that has been commercially developed for the analysis of secreted proteins in serum and blood plasma. This technology can translate protein information into actionable knowledge by linking protein-specific antibodies to DNA-encoded tags. It offers high specificity and sensitivity (sub-pg/ml), enabling high multiplex assays with coverage across a broad dynamic range (~9 log), while consuming minimal quantities of sample. In PEA, matched pairs of oligonucleotide-labeled antibodies bind to their target antigens in a pairwise manner. Upon antibody binding, the matched oligonucleotides are brought into proximity, and using a DNA polymerase a PCR target sequence is created, amplified, detected, and quantified. This downstream process analysis is usually carried out by qPCR or by techniques such as next-generation sequencing (NGS).
The above are all recent technologies, and the coming years will likely see continued growth in the development and application of affinity-based proteomic approaches. The use of probes that target diverse enzyme families has led to a range of applications for activity-based proteomics, in the same way that linking proteins to proteins has provided information about the interactome and protein-DNA interactions have provided further knowledge about cell processes. Therefore, as in other proteomics approaches, comparison of enzyme activity profiles and protein-protein or protein-DNA interactions (based on antibody precipitation) in healthy versus diseased samples can aid the identification of novel biomarkers and drug targets. The results obtained to date show that affinity-based proteomics offer great promise as imaging tools for disease diagnosis and preclinical and clinical testing of therapeutic agents in vivo.
We have also included a new study in table 1 in which the authors used affinity-based methods applied to IMDs, in this case Duchenne muscular dystrophy.
Duchenne muscular dystrophy (DMD; OMIM310200) is a rare X-linked genetic disease with an incidence rate of 1:5,000. DMD is caused by frame-disrupting mutations in the gene encoding dystrophin, resulting in loss of dystrophin [90]. Affected boys typically present clinical signs in the first few years of life, with features suggestive of muscle weakness and often with global developmental delay. Progressive muscle weakness leads to loss of ambulation by the age of 10, and if untreated, to fatal cardio respiratory insufficiency by late adolescence. Establishing a correct diagnosis in dystrophinopathies requires a multidisciplinary approach involving pediatricians, geneticists, and neurologists to define the severity of the clinical phenotype by means of genetic, enzymatic, and immunohistochemical tests [90]. The application of proteomics to the study of dystrophinopathies could facilitate both diagnosis and treatment. In 2014 Ayoglu et al. [36] explored the possibility of identifying circulating candidate protein markers in rare diseases applying an affinity-based proteomics approach to generate a proteomic profile in blood samples from muscular dystrophy patients versus controls. The authors used an antibody bead array platform with 384 antibodies. Based on the results obtained from analysis of both serum and plasma, they identified 11 proteins associated with muscular dystrophy, of which 4 were upregulated in blood from muscular dystrophy patients: carbonic anhydrase III (CA3), myosin light chain 3 (MYL3), mitochondrial malate dehydrogenase 2 (MDH2), and electron transfer flavoprotein A (ETFA). These interesting data could aid the development of new clinical tools for management of dystrophinopathies.
The general description about proteomics methods could be revised cutting (or only mentioning) 2D-PAGE which is a relatively old technique, while including methods such as high multiplexed protein profiling using Proximity Extension Assays (PEA) or Somascan.
A few examples of possibly relevant studies are:
- Cells. 2022 Mar 7;11(5):913. doi: 10.3390/cells11050913.
Hongge Wang et al. Evaluation of Neurofilament Light Chain as a Biomarker of Neurodegeneration in X-Linked Childhood Cerebral Adrenoleukodystrophy
- Blood Adv. 2021 Aug 24;5(16):3092-3101. doi:10.1182/bloodadvances.2020003824.
Brigitte T.A.Long-term effect of hematopoietic cell transplantation on systemic inflammation in patients with mucopolysaccharidoses
We appreciate the reviewer’s comments. We have removed the 2d-PAGE paragraph and included discussion of the PEA technique in section 2.1.4, as referred to in the previous response. The two examples of this technique applied to the study of IMDs were included in table 1 and discussed in the text as we indicated below.
In 2020 van den Broek et al.[71] investigated systemic inflammation in untreated MPS I patients and evaluated the effect of HCT (hematopoietic cell transplantation) on systemic inflammation. The authors used dried blood spot samples from patients with HCT-treated MPS using a OLINK Proseek Multiplex inflammation platform. In total, 92 markers of the OLINK inflammation panel were measured in MPS patients compared with those of age-matched control subjects. The authors observed normal leukocyte enzyme activity levels in 93% of patients post-HCT. Moreover, pretransplant samples showed clear differences between patients and controls. The protein markers that distinguished control subjects from patients pre-HCT were mainly pro-inflammatory (50%) or related to bone homeostasis and extracellular matrix degradation (33%). After 10 years of follow-up, only 5 markers (receptor activator of nuclear factor kappa-B ligand, osteoprotegerin, axis inhibition protein 1 [AXIN1], stem cell factor, and Fms-related tyrosine kinase 3 ligand) remained significantly increased, with a large fold-change difference between MPS I patients and control subjects.
In 2022 Wang et al.[79] used the OLINK Proximity Extension Assay to analyze the cerebral spinal fluid (CSF) of young males with CALD, and performed a comparative analysis using plasma samples. Using the Target 96 Neuro Exploratory panel they found that levels of 5 proteins were significantly increased in CSF. Only for neurofilament light chain (NfL) was a significant correlation observed between CSF and plasma samples. Young males with CALD showed a 11.3-fold increase in plasma NfL compared with controls. Moreover, 9 of 11 young males with CALD who underwent HCT showed a decrease in plasma NfL levels after 1 year of treatment compared with pre-HCT levels. The authors concluded that plasma NfL could constitute a useful determinant of outcomes in CALD
Minor Comments: Figure 1. The sample type should be above the two blocks "Bottom-up" and "Top down" because in the current way it is confusing.
We agree with the reviewer and have edited the figures accordingly.
Figure 4. I would specify the difference between untargeted DIA (eg SWATH) and targeted DIA (SRM/MRM and PRM).
We agree with the reviewer, we change figure 4 adding untargeted DIA for SWATH and targeted DIA for SRM/MRM and PRM. Moreover, the following text has been added to the figure legend:
Figure 4: DDA and DIA mode in proteomic analysis
In DDA mode, extracted proteins are digested and directly analyzed by single-shot DDA. The acquired data is searched against a database of known protein sequences and further processed using diverse software tools. In DIA mode two distinct forms of proteomic analysis can be performed: SWATH-MS (untargeted DIA) and SRM/MRM (targeted DIA). In SWATH-MS extracted proteins are digested and directly analyzed by single-shot analysis in order to generate the spectral library. Once the library is generated a multi-window running method is applied to individual samples: wide isolation windows span the entire MS1 m/z range and all precursor ions in the library that are found in each isolation window are fragmented in MS2. All proteins found in the library are quantified (untargeted) and all samples are processed using data analysis software to obtain the quantitative data. In MRM and SRM, the precursor ions to be analyzed are fixed (targeted) by the user, and only these are fragmented in MS2.
Figure 3 and Figure 4. When talking about targeted MS or quantitative MS the authors should mention that methods such as SRM/MRM and PRM are not simply label free, but they can be quantitative because they imply the use of an internal standard (labeled peptide), while methods based on isobaric or chemical labeling remain semiquantitative.
We agree with the reviewer: it is prudent to emphasize this important difference. The following text has been included at the end of point 2.1.3:
It should be noted that methods such as MRM/SRM/PRM-MS allow for quantification of absolute protein levels. Other previously described methods such as label-based (e.g. ITRAQ, SILAC) and non-label-based methods (e.g. SWATH) are semi quantitative methods that only indicate the fold change in protein levels between two conditions.
Targeted MS/MS allows absolute quantification of targeted proteins using isotopically labelled peptide standards (AQUA) [30–32] or protein standard absolute quantification (PSAQ) [31,33,34]. Using isotope standards simplifies sample preparation and minimizes variation between mass spectrometry devices, thereby increasing the reliability and accuracy of results.
In the review "Proteomics in Inherited Metabolic Disorders" Maria del Pilar Chantada-Vázquez and collegues describe the application of proteomics methods to the study of Inherited Metabolic Disorders (IMD).
The manuscript is well written and comprehensive, however my suggestion is to either change the title to "Mass Spectrometry (MS) in IMD" or to include in the discussion studies based on Affinity Proteomics as well as MS.
We appreciate the comment. We agree with the reviewer and have included a new subsection in section 2.1 Qualitative and Quantitative Proteomics
2.1.4 Affinity-based (probes and antibodies) proteomics approaches [35–38]
Affinity-based proteomics is a relatively new field of proteomics that seeks to characterize protein activity and protein-protein interaction. This method also allows monitoring of the functional regulation of enzymes in complex proteomes. This technology utilizes small molecule activity-based probes (APBs) or protein antibodies. The probe molecules covalently modify the enzyme active site and allow detection and affinity purification of a target enzyme population. Affinity-based antibodies are mainly generated using microarrays or directly by antibody immunoprecipitation of the whole sample. After affinity purification (pulldown based on probes or antibodies), substrates and their exact cleavage sites are identified by mass spectrometry. Moreover, cleavage sites can be quantitatively evaluated by incorporation of quantitative proteomic techniques such as iTRAQ and SILAC [39]. Thus, this technology is highly selective and can be used for analysis of complex samples such as biological fluids, cell lysates, intact cells, and even whole organisms, and can therefore be applied to the study of IMDs.
All activity-based probes share a similar structure[35,39]. They have elements required for targeting, modification, and detection of labeled proteins. Structurally, those elements can be divided into the reactive group, linker, and tag. The reactive functional group provides a covalent attachment to the catalytic residue of the enzyme active site. The linker function separates the reactive functional group from the tag. Finally, the tag enables visualization and/or purification of labeled proteins.
For the antibody microarray, the data set correlates directly with the number of antibodies included on the array and the range of their specificities [37].
Technologies that reveal the most abundant proteins found in certain samples types (e.g. serum) are crucial for the development of biomarkers that can be used as measurable indicators of disease severity and progression, patient stratification, and drug development. Proximity Extension Assay (PEA) is a novel approach based in the interaction between protein antibodies and DNA [38] that has been commercially developed for the analysis of secreted proteins in serum and blood plasma. This technology can translate protein information into actionable knowledge by linking protein-specific antibodies to DNA-encoded tags. It offers high specificity and sensitivity (sub-pg/ml), enabling high multiplex assays with coverage across a broad dynamic range (~9 log), while consuming minimal quantities of sample. In PEA, matched pairs of oligonucleotide-labeled antibodies bind to their target antigens in a pairwise manner. Upon antibody binding, the matched oligonucleotides are brought into proximity, and using a DNA polymerase a PCR target sequence is created, amplified, detected, and quantified. This downstream process analysis is usually carried out by qPCR or by techniques such as next-generation sequencing (NGS).
The above are all recent technologies, and the coming years will likely see continued growth in the development and application of affinity-based proteomic approaches. The use of probes that target diverse enzyme families has led to a range of applications for activity-based proteomics, in the same way that linking proteins to proteins has provided information about the interactome and protein-DNA interactions have provided further knowledge about cell processes. Therefore, as in other proteomics approaches, comparison of enzyme activity profiles and protein-protein or protein-DNA interactions (based on antibody precipitation) in healthy versus diseased samples can aid the identification of novel biomarkers and drug targets. The results obtained to date show that affinity-based proteomics offer great promise as imaging tools for disease diagnosis and preclinical and clinical testing of therapeutic agents in vivo.
We have also included a new study in table 1 in which the authors used affinity-based methods applied to IMDs, in this case Duchenne muscular dystrophy.
Duchenne muscular dystrophy (DMD; OMIM310200) is a rare X-linked genetic disease with an incidence rate of 1:5,000. DMD is caused by frame-disrupting mutations in the gene encoding dystrophin, resulting in loss of dystrophin [90]. Affected boys typically present clinical signs in the first few years of life, with features suggestive of muscle weakness and often with global developmental delay. Progressive muscle weakness leads to loss of ambulation by the age of 10, and if untreated, to fatal cardio respiratory insufficiency by late adolescence. Establishing a correct diagnosis in dystrophinopathies requires a multidisciplinary approach involving pediatricians, geneticists, and neurologists to define the severity of the clinical phenotype by means of genetic, enzymatic, and immunohistochemical tests [90]. The application of proteomics to the study of dystrophinopathies could facilitate both diagnosis and treatment. In 2014 Ayoglu et al. [36] explored the possibility of identifying circulating candidate protein markers in rare diseases applying an affinity-based proteomics approach to generate a proteomic profile in blood samples from muscular dystrophy patients versus controls. The authors used an antibody bead array platform with 384 antibodies. Based on the results obtained from analysis of both serum and plasma, they identified 11 proteins associated with muscular dystrophy, of which 4 were upregulated in blood from muscular dystrophy patients: carbonic anhydrase III (CA3), myosin light chain 3 (MYL3), mitochondrial malate dehydrogenase 2 (MDH2), and electron transfer flavoprotein A (ETFA). These interesting data could aid the development of new clinical tools for management of dystrophinopathies.
The general description about proteomics methods could be revised cutting (or only mentioning) 2D-PAGE which is a relatively old technique, while including methods such as high multiplexed protein profiling using Proximity Extension Assays (PEA) or Somascan.
A few examples of possibly relevant studies are:
- Cells. 2022 Mar 7;11(5):913. doi: 10.3390/cells11050913.
Hongge Wang et al. Evaluation of Neurofilament Light Chain as a Biomarker of Neurodegeneration in X-Linked Childhood Cerebral Adrenoleukodystrophy
- Blood Adv. 2021 Aug 24;5(16):3092-3101. doi:10.1182/bloodadvances.2020003824.
Brigitte T.A.Long-term effect of hematopoietic cell transplantation on systemic inflammation in patients with mucopolysaccharidoses
We appreciate the reviewer’s comments. We have removed the 2d-PAGE paragraph and included discussion of the PEA technique in section 2.1.4, as referred to in the previous response. The two examples of this technique applied to the study of IMDs were included in table 1 and discussed in the text as we indicated below.
In 2020 van den Broek et al.[71] investigated systemic inflammation in untreated MPS I patients and evaluated the effect of HCT (hematopoietic cell transplantation) on systemic inflammation. The authors used dried blood spot samples from patients with HCT-treated MPS using a OLINK Proseek Multiplex inflammation platform. In total, 92 markers of the OLINK inflammation panel were measured in MPS patients compared with those of age-matched control subjects. The authors observed normal leukocyte enzyme activity levels in 93% of patients post-HCT. Moreover, pretransplant samples showed clear differences between patients and controls. The protein markers that distinguished control subjects from patients pre-HCT were mainly pro-inflammatory (50%) or related to bone homeostasis and extracellular matrix degradation (33%). After 10 years of follow-up, only 5 markers (receptor activator of nuclear factor kappa-B ligand, osteoprotegerin, axis inhibition protein 1 [AXIN1], stem cell factor, and Fms-related tyrosine kinase 3 ligand) remained significantly increased, with a large fold-change difference between MPS I patients and control subjects.
In 2022 Wang et al.[79] used the OLINK Proximity Extension Assay to analyze the cerebral spinal fluid (CSF) of young males with CALD, and performed a comparative analysis using plasma samples. Using the Target 96 Neuro Exploratory panel they found that levels of 5 proteins were significantly increased in CSF. Only for neurofilament light chain (NfL) was a significant correlation observed between CSF and plasma samples. Young males with CALD showed a 11.3-fold increase in plasma NfL compared with controls. Moreover, 9 of 11 young males with CALD who underwent HCT showed a decrease in plasma NfL levels after 1 year of treatment compared with pre-HCT levels. The authors concluded that plasma NfL could constitute a useful determinant of outcomes in CALD
Minor Comments: Figure 1. The sample type should be above the two blocks "Bottom-up" and "Top down" because in the current way it is confusing.
We agree with the reviewer and have edited the figures accordingly.
Figure 4. I would specify the difference between untargeted DIA (eg SWATH) and targeted DIA (SRM/MRM and PRM).
We agree with the reviewer, we change figure 4 adding untargeted DIA for SWATH and targeted DIA for SRM/MRM and PRM. Moreover, the following text has been added to the figure legend:
Figure 4: DDA and DIA mode in proteomic analysis
In DDA mode, extracted proteins are digested and directly analyzed by single-shot DDA. The acquired data is searched against a database of known protein sequences and further processed using diverse software tools. In DIA mode two distinct forms of proteomic analysis can be performed: SWATH-MS (untargeted DIA) and SRM/MRM (targeted DIA). In SWATH-MS extracted proteins are digested and directly analyzed by single-shot analysis in order to generate the spectral library. Once the library is generated a multi-window running method is applied to individual samples: wide isolation windows span the entire MS1 m/z range and all precursor ions in the library that are found in each isolation window are fragmented in MS2. All proteins found in the library are quantified (untargeted) and all samples are processed using data analysis software to obtain the quantitative data. In MRM and SRM, the precursor ions to be analyzed are fixed (targeted) by the user, and only these are fragmented in MS2.
Figure 3 and Figure 4. When talking about targeted MS or quantitative MS the authors should mention that methods such as SRM/MRM and PRM are not simply label free, but they can be quantitative because they imply the use of an internal standard (labeled peptide), while methods based on isobaric or chemical labeling remain semiquantitative.
We agree with the reviewer: it is prudent to emphasize this important difference. The following text has been included at the end of point 2.1.3:
It should be noted that methods such as MRM/SRM/PRM-MS allow for quantification of absolute protein levels. Other previously described methods such as label-based (e.g. ITRAQ, SILAC) and non-label-based methods (e.g. SWATH) are semi quantitative methods that only indicate the fold change in protein levels between two conditions.
Targeted MS/MS allows absolute quantification of targeted proteins using isotopically labelled peptide standards (AQUA) [30–32] or protein standard absolute quantification (PSAQ) [31,33,34]. Using isotope standards simplifies sample preparation and minimizes variation between mass spectrometry devices, thereby increasing the reliability and accuracy of results.
In the review "Proteomics in Inherited Metabolic Disorders" Maria del Pilar Chantada-Vázquez and collegues describe the application of proteomics methods to the study of Inherited Metabolic Disorders (IMD).
The manuscript is well written and comprehensive, however my suggestion is to either change the title to "Mass Spectrometry (MS) in IMD" or to include in the discussion studies based on Affinity Proteomics as well as MS.
We appreciate the comment. We agree with the reviewer and have included a new subsection in section 2.1 Qualitative and Quantitative Proteomics
2.1.4 Affinity-based (probes and antibodies) proteomics approaches [35–38]
Affinity-based proteomics is a relatively new field of proteomics that seeks to characterize protein activity and protein-protein interaction. This method also allows monitoring of the functional regulation of enzymes in complex proteomes. This technology utilizes small molecule activity-based probes (APBs) or protein antibodies. The probe molecules covalently modify the enzyme active site and allow detection and affinity purification of a target enzyme population. Affinity-based antibodies are mainly generated using microarrays or directly by antibody immunoprecipitation of the whole sample. After affinity purification (pulldown based on probes or antibodies), substrates and their exact cleavage sites are identified by mass spectrometry. Moreover, cleavage sites can be quantitatively evaluated by incorporation of quantitative proteomic techniques such as iTRAQ and SILAC [39]. Thus, this technology is highly selective and can be used for analysis of complex samples such as biological fluids, cell lysates, intact cells, and even whole organisms, and can therefore be applied to the study of IMDs.
All activity-based probes share a similar structure[35,39]. They have elements required for targeting, modification, and detection of labeled proteins. Structurally, those elements can be divided into the reactive group, linker, and tag. The reactive functional group provides a covalent attachment to the catalytic residue of the enzyme active site. The linker function separates the reactive functional group from the tag. Finally, the tag enables visualization and/or purification of labeled proteins.
For the antibody microarray, the data set correlates directly with the number of antibodies included on the array and the range of their specificities [37].
Technologies that reveal the most abundant proteins found in certain samples types (e.g. serum) are crucial for the development of biomarkers that can be used as measurable indicators of disease severity and progression, patient stratification, and drug development. Proximity Extension Assay (PEA) is a novel approach based in the interaction between protein antibodies and DNA [38] that has been commercially developed for the analysis of secreted proteins in serum and blood plasma. This technology can translate protein information into actionable knowledge by linking protein-specific antibodies to DNA-encoded tags. It offers high specificity and sensitivity (sub-pg/ml), enabling high multiplex assays with coverage across a broad dynamic range (~9 log), while consuming minimal quantities of sample. In PEA, matched pairs of oligonucleotide-labeled antibodies bind to their target antigens in a pairwise manner. Upon antibody binding, the matched oligonucleotides are brought into proximity, and using a DNA polymerase a PCR target sequence is created, amplified, detected, and quantified. This downstream process analysis is usually carried out by qPCR or by techniques such as next-generation sequencing (NGS).
The above are all recent technologies, and the coming years will likely see continued growth in the development and application of affinity-based proteomic approaches. The use of probes that target diverse enzyme families has led to a range of applications for activity-based proteomics, in the same way that linking proteins to proteins has provided information about the interactome and protein-DNA interactions have provided further knowledge about cell processes. Therefore, as in other proteomics approaches, comparison of enzyme activity profiles and protein-protein or protein-DNA interactions (based on antibody precipitation) in healthy versus diseased samples can aid the identification of novel biomarkers and drug targets. The results obtained to date show that affinity-based proteomics offer great promise as imaging tools for disease diagnosis and preclinical and clinical testing of therapeutic agents in vivo.
We have also included a new study in table 1 in which the authors used affinity-based methods applied to IMDs, in this case Duchenne muscular dystrophy.
Duchenne muscular dystrophy (DMD; OMIM310200) is a rare X-linked genetic disease with an incidence rate of 1:5,000. DMD is caused by frame-disrupting mutations in the gene encoding dystrophin, resulting in loss of dystrophin [90]. Affected boys typically present clinical signs in the first few years of life, with features suggestive of muscle weakness and often with global developmental delay. Progressive muscle weakness leads to loss of ambulation by the age of 10, and if untreated, to fatal cardio respiratory insufficiency by late adolescence. Establishing a correct diagnosis in dystrophinopathies requires a multidisciplinary approach involving pediatricians, geneticists, and neurologists to define the severity of the clinical phenotype by means of genetic, enzymatic, and immunohistochemical tests [90]. The application of proteomics to the study of dystrophinopathies could facilitate both diagnosis and treatment. In 2014 Ayoglu et al. [36] explored the possibility of identifying circulating candidate protein markers in rare diseases applying an affinity-based proteomics approach to generate a proteomic profile in blood samples from muscular dystrophy patients versus controls. The authors used an antibody bead array platform with 384 antibodies. Based on the results obtained from analysis of both serum and plasma, they identified 11 proteins associated with muscular dystrophy, of which 4 were upregulated in blood from muscular dystrophy patients: carbonic anhydrase III (CA3), myosin light chain 3 (MYL3), mitochondrial malate dehydrogenase 2 (MDH2), and electron transfer flavoprotein A (ETFA). These interesting data could aid the development of new clinical tools for management of dystrophinopathies.
The general description about proteomics methods could be revised cutting (or only mentioning) 2D-PAGE which is a relatively old technique, while including methods such as high multiplexed protein profiling using Proximity Extension Assays (PEA) or Somascan.
A few examples of possibly relevant studies are:
- Cells. 2022 Mar 7;11(5):913. doi: 10.3390/cells11050913.
Hongge Wang et al. Evaluation of Neurofilament Light Chain as a Biomarker of Neurodegeneration in X-Linked Childhood Cerebral Adrenoleukodystrophy
- Blood Adv. 2021 Aug 24;5(16):3092-3101. doi:10.1182/bloodadvances.2020003824.
Brigitte T.A.Long-term effect of hematopoietic cell transplantation on systemic inflammation in patients with mucopolysaccharidoses
We appreciate the reviewer’s comments. We have removed the 2d-PAGE paragraph and included discussion of the PEA technique in section 2.1.4, as referred to in the previous response. The two examples of this technique applied to the study of IMDs were included in table 1 and discussed in the text as we indicated below.
In 2020 van den Broek et al.[71] investigated systemic inflammation in untreated MPS I patients and evaluated the effect of HCT (hematopoietic cell transplantation) on systemic inflammation. The authors used dried blood spot samples from patients with HCT-treated MPS using a OLINK Proseek Multiplex inflammation platform. In total, 92 markers of the OLINK inflammation panel were measured in MPS patients compared with those of age-matched control subjects. The authors observed normal leukocyte enzyme activity levels in 93% of patients post-HCT. Moreover, pretransplant samples showed clear differences between patients and controls. The protein markers that distinguished control subjects from patients pre-HCT were mainly pro-inflammatory (50%) or related to bone homeostasis and extracellular matrix degradation (33%). After 10 years of follow-up, only 5 markers (receptor activator of nuclear factor kappa-B ligand, osteoprotegerin, axis inhibition protein 1 [AXIN1], stem cell factor, and Fms-related tyrosine kinase 3 ligand) remained significantly increased, with a large fold-change difference between MPS I patients and control subjects.
In 2022 Wang et al.[79] used the OLINK Proximity Extension Assay to analyze the cerebral spinal fluid (CSF) of young males with CALD, and performed a comparative analysis using plasma samples. Using the Target 96 Neuro Exploratory panel they found that levels of 5 proteins were significantly increased in CSF. Only for neurofilament light chain (NfL) was a significant correlation observed between CSF and plasma samples. Young males with CALD showed a 11.3-fold increase in plasma NfL compared with controls. Moreover, 9 of 11 young males with CALD who underwent HCT showed a decrease in plasma NfL levels after 1 year of treatment compared with pre-HCT levels. The authors concluded that plasma NfL could constitute a useful determinant of outcomes in CALD
Minor Comments: Figure 1. The sample type should be above the two blocks "Bottom-up" and "Top down" because in the current way it is confusing.
We agree with the reviewer and have edited the figures accordingly.
Figure 4. I would specify the difference between untargeted DIA (eg SWATH) and targeted DIA (SRM/MRM and PRM).
We agree with the reviewer, we change figure 4 adding untargeted DIA for SWATH and targeted DIA for SRM/MRM and PRM. Moreover, the following text has been added to the figure legend:
Figure 4: DDA and DIA mode in proteomic analysis
In DDA mode, extracted proteins are digested and directly analyzed by single-shot DDA. The acquired data is searched against a database of known protein sequences and further processed using diverse software tools. In DIA mode two distinct forms of proteomic analysis can be performed: SWATH-MS (untargeted DIA) and SRM/MRM (targeted DIA). In SWATH-MS extracted proteins are digested and directly analyzed by single-shot analysis in order to generate the spectral library. Once the library is generated a multi-window running method is applied to individual samples: wide isolation windows span the entire MS1 m/z range and all precursor ions in the library that are found in each isolation window are fragmented in MS2. All proteins found in the library are quantified (untargeted) and all samples are processed using data analysis software to obtain the quantitative data. In MRM and SRM, the precursor ions to be analyzed are fixed (targeted) by the user, and only these are fragmented in MS2.
Figure 3 and Figure 4. When talking about targeted MS or quantitative MS the authors should mention that methods such as SRM/MRM and PRM are not simply label free, but they can be quantitative because they imply the use of an internal standard (labeled peptide), while methods based on isobaric or chemical labeling remain semiquantitative.
We agree with the reviewer: it is prudent to emphasize this important difference. The following text has been included at the end of point 2.1.3:
It should be noted that methods such as MRM/SRM/PRM-MS allow for quantification of absolute protein levels. Other previously described methods such as label-based (e.g. ITRAQ, SILAC) and non-label-based methods (e.g. SWATH) are semi quantitative methods that only indicate the fold change in protein levels between two conditions.
Targeted MS/MS allows absolute quantification of targeted proteins using isotopically labelled peptide standards (AQUA) [30–32] or protein standard absolute quantification (PSAQ) [31,33,34]. Using isotope standards simplifies sample preparation and minimizes variation between mass spectrometry devices, thereby increasing the reliability and accuracy of results.
In the review "Proteomics in Inherited Metabolic Disorders" Maria del Pilar Chantada-Vázquez and collegues describe the application of proteomics methods to the study of Inherited Metabolic Disorders (IMD).
The manuscript is well written and comprehensive, however my suggestion is to either change the title to "Mass Spectrometry (MS) in IMD" or to include in the discussion studies based on Affinity Proteomics as well as MS.
We appreciate the comment. We agree with the reviewer and have included a new subsection in section 2.1 Qualitative and Quantitative Proteomics
2.1.4 Affinity-based (probes and antibodies) proteomics approaches [35–38]
Affinity-based proteomics is a relatively new field of proteomics that seeks to characterize protein activity and protein-protein interaction. This method also allows monitoring of the functional regulation of enzymes in complex proteomes. This technology utilizes small molecule activity-based probes (APBs) or protein antibodies. The probe molecules covalently modify the enzyme active site and allow detection and affinity purification of a target enzyme population. Affinity-based antibodies are mainly generated using microarrays or directly by antibody immunoprecipitation of the whole sample. After affinity purification (pulldown based on probes or antibodies), substrates and their exact cleavage sites are identified by mass spectrometry. Moreover, cleavage sites can be quantitatively evaluated by incorporation of quantitative proteomic techniques such as iTRAQ and SILAC [39]. Thus, this technology is highly selective and can be used for analysis of complex samples such as biological fluids, cell lysates, intact cells, and even whole organisms, and can therefore be applied to the study of IMDs.
All activity-based probes share a similar structure[35,39]. They have elements required for targeting, modification, and detection of labeled proteins. Structurally, those elements can be divided into the reactive group, linker, and tag. The reactive functional group provides a covalent attachment to the catalytic residue of the enzyme active site. The linker function separates the reactive functional group from the tag. Finally, the tag enables visualization and/or purification of labeled proteins.
For the antibody microarray, the data set correlates directly with the number of antibodies included on the array and the range of their specificities [37].
Technologies that reveal the most abundant proteins found in certain samples types (e.g. serum) are crucial for the development of biomarkers that can be used as measurable indicators of disease severity and progression, patient stratification, and drug development. Proximity Extension Assay (PEA) is a novel approach based in the interaction between protein antibodies and DNA [38] that has been commercially developed for the analysis of secreted proteins in serum and blood plasma. This technology can translate protein information into actionable knowledge by linking protein-specific antibodies to DNA-encoded tags. It offers high specificity and sensitivity (sub-pg/ml), enabling high multiplex assays with coverage across a broad dynamic range (~9 log), while consuming minimal quantities of sample. In PEA, matched pairs of oligonucleotide-labeled antibodies bind to their target antigens in a pairwise manner. Upon antibody binding, the matched oligonucleotides are brought into proximity, and using a DNA polymerase a PCR target sequence is created, amplified, detected, and quantified. This downstream process analysis is usually carried out by qPCR or by techniques such as next-generation sequencing (NGS).
The above are all recent technologies, and the coming years will likely see continued growth in the development and application of affinity-based proteomic approaches. The use of probes that target diverse enzyme families has led to a range of applications for activity-based proteomics, in the same way that linking proteins to proteins has provided information about the interactome and protein-DNA interactions have provided further knowledge about cell processes. Therefore, as in other proteomics approaches, comparison of enzyme activity profiles and protein-protein or protein-DNA interactions (based on antibody precipitation) in healthy versus diseased samples can aid the identification of novel biomarkers and drug targets. The results obtained to date show that affinity-based proteomics offer great promise as imaging tools for disease diagnosis and preclinical and clinical testing of therapeutic agents in vivo.
We have also included a new study in table 1 in which the authors used affinity-based methods applied to IMDs, in this case Duchenne muscular dystrophy.
Duchenne muscular dystrophy (DMD; OMIM310200) is a rare X-linked genetic disease with an incidence rate of 1:5,000. DMD is caused by frame-disrupting mutations in the gene encoding dystrophin, resulting in loss of dystrophin [90]. Affected boys typically present clinical signs in the first few years of life, with features suggestive of muscle weakness and often with global developmental delay. Progressive muscle weakness leads to loss of ambulation by the age of 10, and if untreated, to fatal cardio respiratory insufficiency by late adolescence. Establishing a correct diagnosis in dystrophinopathies requires a multidisciplinary approach involving pediatricians, geneticists, and neurologists to define the severity of the clinical phenotype by means of genetic, enzymatic, and immunohistochemical tests [90]. The application of proteomics to the study of dystrophinopathies could facilitate both diagnosis and treatment. In 2014 Ayoglu et al. [36] explored the possibility of identifying circulating candidate protein markers in rare diseases applying an affinity-based proteomics approach to generate a proteomic profile in blood samples from muscular dystrophy patients versus controls. The authors used an antibody bead array platform with 384 antibodies. Based on the results obtained from analysis of both serum and plasma, they identified 11 proteins associated with muscular dystrophy, of which 4 were upregulated in blood from muscular dystrophy patients: carbonic anhydrase III (CA3), myosin light chain 3 (MYL3), mitochondrial malate dehydrogenase 2 (MDH2), and electron transfer flavoprotein A (ETFA). These interesting data could aid the development of new clinical tools for management of dystrophinopathies.
The general description about proteomics methods could be revised cutting (or only mentioning) 2D-PAGE which is a relatively old technique, while including methods such as high multiplexed protein profiling using Proximity Extension Assays (PEA) or Somascan.
A few examples of possibly relevant studies are:
- Cells. 2022 Mar 7;11(5):913. doi: 10.3390/cells11050913.
Hongge Wang et al. Evaluation of Neurofilament Light Chain as a Biomarker of Neurodegeneration in X-Linked Childhood Cerebral Adrenoleukodystrophy
- Blood Adv. 2021 Aug 24;5(16):3092-3101. doi:10.1182/bloodadvances.2020003824.
Brigitte T.A.Long-term effect of hematopoietic cell transplantation on systemic inflammation in patients with mucopolysaccharidoses
We appreciate the reviewer’s comments. We have removed the 2d-PAGE paragraph and included discussion of the PEA technique in section 2.1.4, as referred to in the previous response. The two examples of this technique applied to the study of IMDs were included in table 1 and discussed in the text as we indicated below.
In 2020 van den Broek et al.[71] investigated systemic inflammation in untreated MPS I patients and evaluated the effect of HCT (hematopoietic cell transplantation) on systemic inflammation. The authors used dried blood spot samples from patients with HCT-treated MPS using a OLINK Proseek Multiplex inflammation platform. In total, 92 markers of the OLINK inflammation panel were measured in MPS patients compared with those of age-matched control subjects. The authors observed normal leukocyte enzyme activity levels in 93% of patients post-HCT. Moreover, pretransplant samples showed clear differences between patients and controls. The protein markers that distinguished control subjects from patients pre-HCT were mainly pro-inflammatory (50%) or related to bone homeostasis and extracellular matrix degradation (33%). After 10 years of follow-up, only 5 markers (receptor activator of nuclear factor kappa-B ligand, osteoprotegerin, axis inhibition protein 1 [AXIN1], stem cell factor, and Fms-related tyrosine kinase 3 ligand) remained significantly increased, with a large fold-change difference between MPS I patients and control subjects.
In 2022 Wang et al.[79] used the OLINK Proximity Extension Assay to analyze the cerebral spinal fluid (CSF) of young males with CALD, and performed a comparative analysis using plasma samples. Using the Target 96 Neuro Exploratory panel they found that levels of 5 proteins were significantly increased in CSF. Only for neurofilament light chain (NfL) was a significant correlation observed between CSF and plasma samples. Young males with CALD showed a 11.3-fold increase in plasma NfL compared with controls. Moreover, 9 of 11 young males with CALD who underwent HCT showed a decrease in plasma NfL levels after 1 year of treatment compared with pre-HCT levels. The authors concluded that plasma NfL could constitute a useful determinant of outcomes in CALD
Minor Comments: Figure 1. The sample type should be above the two blocks "Bottom-up" and "Top down" because in the current way it is confusing.
We agree with the reviewer and have edited the figures accordingly.
Figure 4. I would specify the difference between untargeted DIA (eg SWATH) and targeted DIA (SRM/MRM and PRM).
We agree with the reviewer, we change figure 4 adding untargeted DIA for SWATH and targeted DIA for SRM/MRM and PRM. Moreover, the following text has been added to the figure legend:
Figure 4: DDA and DIA mode in proteomic analysis
In DDA mode, extracted proteins are digested and directly analyzed by single-shot DDA. The acquired data is searched against a database of known protein sequences and further processed using diverse software tools. In DIA mode two distinct forms of proteomic analysis can be performed: SWATH-MS (untargeted DIA) and SRM/MRM (targeted DIA). In SWATH-MS extracted proteins are digested and directly analyzed by single-shot analysis in order to generate the spectral library. Once the library is generated a multi-window running method is applied to individual samples: wide isolation windows span the entire MS1 m/z range and all precursor ions in the library that are found in each isolation window are fragmented in MS2. All proteins found in the library are quantified (untargeted) and all samples are processed using data analysis software to obtain the quantitative data. In MRM and SRM, the precursor ions to be analyzed are fixed (targeted) by the user, and only these are fragmented in MS2.
Figure 3 and Figure 4. When talking about targeted MS or quantitative MS the authors should mention that methods such as SRM/MRM and PRM are not simply label free, but they can be quantitative because they imply the use of an internal standard (labeled peptide), while methods based on isobaric or chemical labeling remain semiquantitative.
We agree with the reviewer: it is prudent to emphasize this important difference. The following text has been included at the end of point 2.1.3:
It should be noted that methods such as MRM/SRM/PRM-MS allow for quantification of absolute protein levels. Other previously described methods such as label-based (e.g. ITRAQ, SILAC) and non-label-based methods (e.g. SWATH) are semi quantitative methods that only indicate the fold change in protein levels between two conditions.
Targeted MS/MS allows absolute quantification of targeted proteins using isotopically labelled peptide standards (AQUA) [30–32] or protein standard absolute quantification (PSAQ) [31,33,34]. Using isotope standards simplifies sample preparation and minimizes variation between mass spectrometry devices, thereby increasing the reliability and accuracy of results.
In the review "Proteomics in Inherited Metabolic Disorders" Maria del Pilar Chantada-Vázquez and collegues describe the application of proteomics methods to the study of Inherited Metabolic Disorders (IMD).
The manuscript is well written and comprehensive, however my suggestion is to either change the title to "Mass Spectrometry (MS) in IMD" or to include in the discussion studies based on Affinity Proteomics as well as MS.
We appreciate the comment. We agree with the reviewer and have included a new subsection in section 2.1 Qualitative and Quantitative Proteomics
2.1.4 Affinity-based (probes and antibodies) proteomics approaches [35–38]
Affinity-based proteomics is a relatively new field of proteomics that seeks to characterize protein activity and protein-protein interaction. This method also allows monitoring of the functional regulation of enzymes in complex proteomes. This technology utilizes small molecule activity-based probes (APBs) or protein antibodies. The probe molecules covalently modify the enzyme active site and allow detection and affinity purification of a target enzyme population. Affinity-based antibodies are mainly generated using microarrays or directly by antibody immunoprecipitation of the whole sample. After affinity purification (pulldown based on probes or antibodies), substrates and their exact cleavage sites are identified by mass spectrometry. Moreover, cleavage sites can be quantitatively evaluated by incorporation of quantitative proteomic techniques such as iTRAQ and SILAC [39]. Thus, this technology is highly selective and can be used for analysis of complex samples such as biological fluids, cell lysates, intact cells, and even whole organisms, and can therefore be applied to the study of IMDs.
All activity-based probes share a similar structure[35,39]. They have elements required for targeting, modification, and detection of labeled proteins. Structurally, those elements can be divided into the reactive group, linker, and tag. The reactive functional group provides a covalent attachment to the catalytic residue of the enzyme active site. The linker function separates the reactive functional group from the tag. Finally, the tag enables visualization and/or purification of labeled proteins.
For the antibody microarray, the data set correlates directly with the number of antibodies included on the array and the range of their specificities [37].
Technologies that reveal the most abundant proteins found in certain samples types (e.g. serum) are crucial for the development of biomarkers that can be used as measurable indicators of disease severity and progression, patient stratification, and drug development. Proximity Extension Assay (PEA) is a novel approach based in the interaction between protein antibodies and DNA [38] that has been commercially developed for the analysis of secreted proteins in serum and blood plasma. This technology can translate protein information into actionable knowledge by linking protein-specific antibodies to DNA-encoded tags. It offers high specificity and sensitivity (sub-pg/ml), enabling high multiplex assays with coverage across a broad dynamic range (~9 log), while consuming minimal quantities of sample. In PEA, matched pairs of oligonucleotide-labeled antibodies bind to their target antigens in a pairwise manner. Upon antibody binding, the matched oligonucleotides are brought into proximity, and using a DNA polymerase a PCR target sequence is created, amplified, detected, and quantified. This downstream process analysis is usually carried out by qPCR or by techniques such as next-generation sequencing (NGS).
The above are all recent technologies, and the coming years will likely see continued growth in the development and application of affinity-based proteomic approaches. The use of probes that target diverse enzyme families has led to a range of applications for activity-based proteomics, in the same way that linking proteins to proteins has provided information about the interactome and protein-DNA interactions have provided further knowledge about cell processes. Therefore, as in other proteomics approaches, comparison of enzyme activity profiles and protein-protein or protein-DNA interactions (based on antibody precipitation) in healthy versus diseased samples can aid the identification of novel biomarkers and drug targets. The results obtained to date show that affinity-based proteomics offer great promise as imaging tools for disease diagnosis and preclinical and clinical testing of therapeutic agents in vivo.
We have also included a new study in table 1 in which the authors used affinity-based methods applied to IMDs, in this case Duchenne muscular dystrophy.
Duchenne muscular dystrophy (DMD; OMIM310200) is a rare X-linked genetic disease with an incidence rate of 1:5,000. DMD is caused by frame-disrupting mutations in the gene encoding dystrophin, resulting in loss of dystrophin [90]. Affected boys typically present clinical signs in the first few years of life, with features suggestive of muscle weakness and often with global developmental delay. Progressive muscle weakness leads to loss of ambulation by the age of 10, and if untreated, to fatal cardio respiratory insufficiency by late adolescence. Establishing a correct diagnosis in dystrophinopathies requires a multidisciplinary approach involving pediatricians, geneticists, and neurologists to define the severity of the clinical phenotype by means of genetic, enzymatic, and immunohistochemical tests [90]. The application of proteomics to the study of dystrophinopathies could facilitate both diagnosis and treatment. In 2014 Ayoglu et al. [36] explored the possibility of identifying circulating candidate protein markers in rare diseases applying an affinity-based proteomics approach to generate a proteomic profile in blood samples from muscular dystrophy patients versus controls. The authors used an antibody bead array platform with 384 antibodies. Based on the results obtained from analysis of both serum and plasma, they identified 11 proteins associated with muscular dystrophy, of which 4 were upregulated in blood from muscular dystrophy patients: carbonic anhydrase III (CA3), myosin light chain 3 (MYL3), mitochondrial malate dehydrogenase 2 (MDH2), and electron transfer flavoprotein A (ETFA). These interesting data could aid the development of new clinical tools for management of dystrophinopathies.
The general description about proteomics methods could be revised cutting (or only mentioning) 2D-PAGE which is a relatively old technique, while including methods such as high multiplexed protein profiling using Proximity Extension Assays (PEA) or Somascan.
A few examples of possibly relevant studies are:
- Cells. 2022 Mar 7;11(5):913. doi: 10.3390/cells11050913.
Hongge Wang et al. Evaluation of Neurofilament Light Chain as a Biomarker of Neurodegeneration in X-Linked Childhood Cerebral Adrenoleukodystrophy
- Blood Adv. 2021 Aug 24;5(16):3092-3101. doi:10.1182/bloodadvances.2020003824.
Brigitte T.A.Long-term effect of hematopoietic cell transplantation on systemic inflammation in patients with mucopolysaccharidoses
We appreciate the reviewer’s comments. We have removed the 2d-PAGE paragraph and included discussion of the PEA technique in section 2.1.4, as referred to in the previous response. The two examples of this technique applied to the study of IMDs were included in table 1 and discussed in the text as we indicated below.
In 2020 van den Broek et al.[71] investigated systemic inflammation in untreated MPS I patients and evaluated the effect of HCT (hematopoietic cell transplantation) on systemic inflammation. The authors used dried blood spot samples from patients with HCT-treated MPS using a OLINK Proseek Multiplex inflammation platform. In total, 92 markers of the OLINK inflammation panel were measured in MPS patients compared with those of age-matched control subjects. The authors observed normal leukocyte enzyme activity levels in 93% of patients post-HCT. Moreover, pretransplant samples showed clear differences between patients and controls. The protein markers that distinguished control subjects from patients pre-HCT were mainly pro-inflammatory (50%) or related to bone homeostasis and extracellular matrix degradation (33%). After 10 years of follow-up, only 5 markers (receptor activator of nuclear factor kappa-B ligand, osteoprotegerin, axis inhibition protein 1 [AXIN1], stem cell factor, and Fms-related tyrosine kinase 3 ligand) remained significantly increased, with a large fold-change difference between MPS I patients and control subjects.
In 2022 Wang et al.[79] used the OLINK Proximity Extension Assay to analyze the cerebral spinal fluid (CSF) of young males with CALD, and performed a comparative analysis using plasma samples. Using the Target 96 Neuro Exploratory panel they found that levels of 5 proteins were significantly increased in CSF. Only for neurofilament light chain (NfL) was a significant correlation observed between CSF and plasma samples. Young males with CALD showed a 11.3-fold increase in plasma NfL compared with controls. Moreover, 9 of 11 young males with CALD who underwent HCT showed a decrease in plasma NfL levels after 1 year of treatment compared with pre-HCT levels. The authors concluded that plasma NfL could constitute a useful determinant of outcomes in CALD
Minor Comments: Figure 1. The sample type should be above the two blocks "Bottom-up" and "Top down" because in the current way it is confusing.
We agree with the reviewer and have edited the figures accordingly.
Figure 4. I would specify the difference between untargeted DIA (eg SWATH) and targeted DIA (SRM/MRM and PRM).
We agree with the reviewer, we change figure 4 adding untargeted DIA for SWATH and targeted DIA for SRM/MRM and PRM. Moreover, the following text has been added to the figure legend:
Figure 4: DDA and DIA mode in proteomic analysis
In DDA mode, extracted proteins are digested and directly analyzed by single-shot DDA. The acquired data is searched against a database of known protein sequences and further processed using diverse software tools. In DIA mode two distinct forms of proteomic analysis can be performed: SWATH-MS (untargeted DIA) and SRM/MRM (targeted DIA). In SWATH-MS extracted proteins are digested and directly analyzed by single-shot analysis in order to generate the spectral library. Once the library is generated a multi-window running method is applied to individual samples: wide isolation windows span the entire MS1 m/z range and all precursor ions in the library that are found in each isolation window are fragmented in MS2. All proteins found in the library are quantified (untargeted) and all samples are processed using data analysis software to obtain the quantitative data. In MRM and SRM, the precursor ions to be analyzed are fixed (targeted) by the user, and only these are fragmented in MS2.
Figure 3 and Figure 4. When talking about targeted MS or quantitative MS the authors should mention that methods such as SRM/MRM and PRM are not simply label free, but they can be quantitative because they imply the use of an internal standard (labeled peptide), while methods based on isobaric or chemical labeling remain semiquantitative.
We agree with the reviewer: it is prudent to emphasize this important difference. The following text has been included at the end of point 2.1.3:
It should be noted that methods such as MRM/SRM/PRM-MS allow for quantification of absolute protein levels. Other previously described methods such as label-based (e.g. ITRAQ, SILAC) and non-label-based methods (e.g. SWATH) are semi quantitative methods that only indicate the fold change in protein levels between two conditions.
Targeted MS/MS allows absolute quantification of targeted proteins using isotopically labelled peptide standards (AQUA) [30–32] or protein standard absolute quantification (PSAQ) [31,33,34]. Using isotope standards simplifies sample preparation and minimizes variation between mass spectrometry devices, thereby increasing the reliability and accuracy of results.
In the review "Proteomics in Inherited Metabolic Disorders" Maria del Pilar Chantada-Vázquez and collegues describe the application of proteomics methods to the study of Inherited Metabolic Disorders (IMD).
The manuscript is well written and comprehensive, however my suggestion is to either change the title to "Mass Spectrometry (MS) in IMD" or to include in the discussion studies based on Affinity Proteomics as well as MS.
We appreciate the comment. We agree with the reviewer and have included a new subsection in section 2.1 Qualitative and Quantitative Proteomics
2.1.4 Affinity-based (probes and antibodies) proteomics approaches [35–38]
Affinity-based proteomics is a relatively new field of proteomics that seeks to characterize protein activity and protein-protein interaction. This method also allows monitoring of the functional regulation of enzymes in complex proteomes. This technology utilizes small molecule activity-based probes (APBs) or protein antibodies. The probe molecules covalently modify the enzyme active site and allow detection and affinity purification of a target enzyme population. Affinity-based antibodies are mainly generated using microarrays or directly by antibody immunoprecipitation of the whole sample. After affinity purification (pulldown based on probes or antibodies), substrates and their exact cleavage sites are identified by mass spectrometry. Moreover, cleavage sites can be quantitatively evaluated by incorporation of quantitative proteomic techniques such as iTRAQ and SILAC [39]. Thus, this technology is highly selective and can be used for analysis of complex samples such as biological fluids, cell lysates, intact cells, and even whole organisms, and can therefore be applied to the study of IMDs.
All activity-based probes share a similar structure[35,39]. They have elements required for targeting, modification, and detection of labeled proteins. Structurally, those elements can be divided into the reactive group, linker, and tag. The reactive functional group provides a covalent attachment to the catalytic residue of the enzyme active site. The linker function separates the reactive functional group from the tag. Finally, the tag enables visualization and/or purification of labeled proteins.
For the antibody microarray, the data set correlates directly with the number of antibodies included on the array and the range of their specificities [37].
Technologies that reveal the most abundant proteins found in certain samples types (e.g. serum) are crucial for the development of biomarkers that can be used as measurable indicators of disease severity and progression, patient stratification, and drug development. Proximity Extension Assay (PEA) is a novel approach based in the interaction between protein antibodies and DNA [38] that has been commercially developed for the analysis of secreted proteins in serum and blood plasma. This technology can translate protein information into actionable knowledge by linking protein-specific antibodies to DNA-encoded tags. It offers high specificity and sensitivity (sub-pg/ml), enabling high multiplex assays with coverage across a broad dynamic range (~9 log), while consuming minimal quantities of sample. In PEA, matched pairs of oligonucleotide-labeled antibodies bind to their target antigens in a pairwise manner. Upon antibody binding, the matched oligonucleotides are brought into proximity, and using a DNA polymerase a PCR target sequence is created, amplified, detected, and quantified. This downstream process analysis is usually carried out by qPCR or by techniques such as next-generation sequencing (NGS).
The above are all recent technologies, and the coming years will likely see continued growth in the development and application of affinity-based proteomic approaches. The use of probes that target diverse enzyme families has led to a range of applications for activity-based proteomics, in the same way that linking proteins to proteins has provided information about the interactome and protein-DNA interactions have provided further knowledge about cell processes. Therefore, as in other proteomics approaches, comparison of enzyme activity profiles and protein-protein or protein-DNA interactions (based on antibody precipitation) in healthy versus diseased samples can aid the identification of novel biomarkers and drug targets. The results obtained to date show that affinity-based proteomics offer great promise as imaging tools for disease diagnosis and preclinical and clinical testing of therapeutic agents in vivo.
We have also included a new study in table 1 in which the authors used affinity-based methods applied to IMDs, in this case Duchenne muscular dystrophy.
Duchenne muscular dystrophy (DMD; OMIM310200) is a rare X-linked genetic disease with an incidence rate of 1:5,000. DMD is caused by frame-disrupting mutations in the gene encoding dystrophin, resulting in loss of dystrophin [90]. Affected boys typically present clinical signs in the first few years of life, with features suggestive of muscle weakness and often with global developmental delay. Progressive muscle weakness leads to loss of ambulation by the age of 10, and if untreated, to fatal cardio respiratory insufficiency by late adolescence. Establishing a correct diagnosis in dystrophinopathies requires a multidisciplinary approach involving pediatricians, geneticists, and neurologists to define the severity of the clinical phenotype by means of genetic, enzymatic, and immunohistochemical tests [90]. The application of proteomics to the study of dystrophinopathies could facilitate both diagnosis and treatment. In 2014 Ayoglu et al. [36] explored the possibility of identifying circulating candidate protein markers in rare diseases applying an affinity-based proteomics approach to generate a proteomic profile in blood samples from muscular dystrophy patients versus controls. The authors used an antibody bead array platform with 384 antibodies. Based on the results obtained from analysis of both serum and plasma, they identified 11 proteins associated with muscular dystrophy, of which 4 were upregulated in blood from muscular dystrophy patients: carbonic anhydrase III (CA3), myosin light chain 3 (MYL3), mitochondrial malate dehydrogenase 2 (MDH2), and electron transfer flavoprotein A (ETFA). These interesting data could aid the development of new clinical tools for management of dystrophinopathies.
The general description about proteomics methods could be revised cutting (or only mentioning) 2D-PAGE which is a relatively old technique, while including methods such as high multiplexed protein profiling using Proximity Extension Assays (PEA) or Somascan.
A few examples of possibly relevant studies are:
- Cells. 2022 Mar 7;11(5):913. doi: 10.3390/cells11050913.
Hongge Wang et al. Evaluation of Neurofilament Light Chain as a Biomarker of Neurodegeneration in X-Linked Childhood Cerebral Adrenoleukodystrophy
- Blood Adv. 2021 Aug 24;5(16):3092-3101. doi:10.1182/bloodadvances.2020003824.
Brigitte T.A.Long-term effect of hematopoietic cell transplantation on systemic inflammation in patients with mucopolysaccharidoses
We appreciate the reviewer’s comments. We have removed the 2d-PAGE paragraph and included discussion of the PEA technique in section 2.1.4, as referred to in the previous response. The two examples of this technique applied to the study of IMDs were included in table 1 and discussed in the text as we indicated below.
In 2020 van den Broek et al.[71] investigated systemic inflammation in untreated MPS I patients and evaluated the effect of HCT (hematopoietic cell transplantation) on systemic inflammation. The authors used dried blood spot samples from patients with HCT-treated MPS using a OLINK Proseek Multiplex inflammation platform. In total, 92 markers of the OLINK inflammation panel were measured in MPS patients compared with those of age-matched control subjects. The authors observed normal leukocyte enzyme activity levels in 93% of patients post-HCT. Moreover, pretransplant samples showed clear differences between patients and controls. The protein markers that distinguished control subjects from patients pre-HCT were mainly pro-inflammatory (50%) or related to bone homeostasis and extracellular matrix degradation (33%). After 10 years of follow-up, only 5 markers (receptor activator of nuclear factor kappa-B ligand, osteoprotegerin, axis inhibition protein 1 [AXIN1], stem cell factor, and Fms-related tyrosine kinase 3 ligand) remained significantly increased, with a large fold-change difference between MPS I patients and control subjects.
In 2022 Wang et al.[79] used the OLINK Proximity Extension Assay to analyze the cerebral spinal fluid (CSF) of young males with CALD, and performed a comparative analysis using plasma samples. Using the Target 96 Neuro Exploratory panel they found that levels of 5 proteins were significantly increased in CSF. Only for neurofilament light chain (NfL) was a significant correlation observed between CSF and plasma samples. Young males with CALD showed a 11.3-fold increase in plasma NfL compared with controls. Moreover, 9 of 11 young males with CALD who underwent HCT showed a decrease in plasma NfL levels after 1 year of treatment compared with pre-HCT levels. The authors concluded that plasma NfL could constitute a useful determinant of outcomes in CALD
Minor Comments: Figure 1. The sample type should be above the two blocks "Bottom-up" and "Top down" because in the current way it is confusing.
We agree with the reviewer and have edited the figures accordingly.
Figure 4. I would specify the difference between untargeted DIA (eg SWATH) and targeted DIA (SRM/MRM and PRM).
We agree with the reviewer, we change figure 4 adding untargeted DIA for SWATH and targeted DIA for SRM/MRM and PRM. Moreover, the following text has been added to the figure legend:
Figure 4: DDA and DIA mode in proteomic analysis
In DDA mode, extracted proteins are digested and directly analyzed by single-shot DDA. The acquired data is searched against a database of known protein sequences and further processed using diverse software tools. In DIA mode two distinct forms of proteomic analysis can be performed: SWATH-MS (untargeted DIA) and SRM/MRM (targeted DIA). In SWATH-MS extracted proteins are digested and directly analyzed by single-shot analysis in order to generate the spectral library. Once the library is generated a multi-window running method is applied to individual samples: wide isolation windows span the entire MS1 m/z range and all precursor ions in the library that are found in each isolation window are fragmented in MS2. All proteins found in the library are quantified (untargeted) and all samples are processed using data analysis software to obtain the quantitative data. In MRM and SRM, the precursor ions to be analyzed are fixed (targeted) by the user, and only these are fragmented in MS2.
Figure 3 and Figure 4. When talking about targeted MS or quantitative MS the authors should mention that methods such as SRM/MRM and PRM are not simply label free, but they can be quantitative because they imply the use of an internal standard (labeled peptide), while methods based on isobaric or chemical labeling remain semiquantitative.
We agree with the reviewer: it is prudent to emphasize this important difference. The following text has been included at the end of point 2.1.3:
It should be noted that methods such as MRM/SRM/PRM-MS allow for quantification of absolute protein levels. Other previously described methods such as label-based (e.g. ITRAQ, SILAC) and non-label-based methods (e.g. SWATH) are semi quantitative methods that only indicate the fold change in protein levels between two conditions.
Targeted MS/MS allows absolute quantification of targeted proteins using isotopically labelled peptide standards (AQUA) [30–32] or protein standard absolute quantification (PSAQ) [31,33,34]. Using isotope standards simplifies sample preparation and minimizes variation between mass spectrometry devices, thereby increasing the reliability and accuracy of results.
In the review "Proteomics in Inherited Metabolic Disorders" Maria del Pilar Chantada-Vázquez and collegues describe the application of proteomics methods to the study of Inherited Metabolic Disorders (IMD).
The manuscript is well written and comprehensive, however my suggestion is to either change the title to "Mass Spectrometry (MS) in IMD" or to include in the discussion studies based on Affinity Proteomics as well as MS.
We appreciate the comment. We agree with the reviewer and have included a new subsection in section 2.1 Qualitative and Quantitative Proteomics
2.1.4 Affinity-based (probes and antibodies) proteomics approaches [35–38]
Affinity-based proteomics is a relatively new field of proteomics that seeks to characterize protein activity and protein-protein interaction. This method also allows monitoring of the functional regulation of enzymes in complex proteomes. This technology utilizes small molecule activity-based probes (APBs) or protein antibodies. The probe molecules covalently modify the enzyme active site and allow detection and affinity purification of a target enzyme population. Affinity-based antibodies are mainly generated using microarrays or directly by antibody immunoprecipitation of the whole sample. After affinity purification (pulldown based on probes or antibodies), substrates and their exact cleavage sites are identified by mass spectrometry. Moreover, cleavage sites can be quantitatively evaluated by incorporation of quantitative proteomic techniques such as iTRAQ and SILAC [39]. Thus, this technology is highly selective and can be used for analysis of complex samples such as biological fluids, cell lysates, intact cells, and even whole organisms, and can therefore be applied to the study of IMDs.
All activity-based probes share a similar structure[35,39]. They have elements required for targeting, modification, and detection of labeled proteins. Structurally, those elements can be divided into the reactive group, linker, and tag. The reactive functional group provides a covalent attachment to the catalytic residue of the enzyme active site. The linker function separates the reactive functional group from the tag. Finally, the tag enables visualization and/or purification of labeled proteins.
For the antibody microarray, the data set correlates directly with the number of antibodies included on the array and the range of their specificities [37].
Technologies that reveal the most abundant proteins found in certain samples types (e.g. serum) are crucial for the development of biomarkers that can be used as measurable indicators of disease severity and progression, patient stratification, and drug development. Proximity Extension Assay (PEA) is a novel approach based in the interaction between protein antibodies and DNA [38] that has been commercially developed for the analysis of secreted proteins in serum and blood plasma. This technology can translate protein information into actionable knowledge by linking protein-specific antibodies to DNA-encoded tags. It offers high specificity and sensitivity (sub-pg/ml), enabling high multiplex assays with coverage across a broad dynamic range (~9 log), while consuming minimal quantities of sample. In PEA, matched pairs of oligonucleotide-labeled antibodies bind to their target antigens in a pairwise manner. Upon antibody binding, the matched oligonucleotides are brought into proximity, and using a DNA polymerase a PCR target sequence is created, amplified, detected, and quantified. This downstream process analysis is usually carried out by qPCR or by techniques such as next-generation sequencing (NGS).
The above are all recent technologies, and the coming years will likely see continued growth in the development and application of affinity-based proteomic approaches. The use of probes that target diverse enzyme families has led to a range of applications for activity-based proteomics, in the same way that linking proteins to proteins has provided information about the interactome and protein-DNA interactions have provided further knowledge about cell processes. Therefore, as in other proteomics approaches, comparison of enzyme activity profiles and protein-protein or protein-DNA interactions (based on antibody precipitation) in healthy versus diseased samples can aid the identification of novel biomarkers and drug targets. The results obtained to date show that affinity-based proteomics offer great promise as imaging tools for disease diagnosis and preclinical and clinical testing of therapeutic agents in vivo.
We have also included a new study in table 1 in which the authors used affinity-based methods applied to IMDs, in this case Duchenne muscular dystrophy.
Duchenne muscular dystrophy (DMD; OMIM310200) is a rare X-linked genetic disease with an incidence rate of 1:5,000. DMD is caused by frame-disrupting mutations in the gene encoding dystrophin, resulting in loss of dystrophin [90]. Affected boys typically present clinical signs in the first few years of life, with features suggestive of muscle weakness and often with global developmental delay. Progressive muscle weakness leads to loss of ambulation by the age of 10, and if untreated, to fatal cardio respiratory insufficiency by late adolescence. Establishing a correct diagnosis in dystrophinopathies requires a multidisciplinary approach involving pediatricians, geneticists, and neurologists to define the severity of the clinical phenotype by means of genetic, enzymatic, and immunohistochemical tests [90]. The application of proteomics to the study of dystrophinopathies could facilitate both diagnosis and treatment. In 2014 Ayoglu et al. [36] explored the possibility of identifying circulating candidate protein markers in rare diseases applying an affinity-based proteomics approach to generate a proteomic profile in blood samples from muscular dystrophy patients versus controls. The authors used an antibody bead array platform with 384 antibodies. Based on the results obtained from analysis of both serum and plasma, they identified 11 proteins associated with muscular dystrophy, of which 4 were upregulated in blood from muscular dystrophy patients: carbonic anhydrase III (CA3), myosin light chain 3 (MYL3), mitochondrial malate dehydrogenase 2 (MDH2), and electron transfer flavoprotein A (ETFA). These interesting data could aid the development of new clinical tools for management of dystrophinopathies.
The general description about proteomics methods could be revised cutting (or only mentioning) 2D-PAGE which is a relatively old technique, while including methods such as high multiplexed protein profiling using Proximity Extension Assays (PEA) or Somascan.
A few examples of possibly relevant studies are:
- Cells. 2022 Mar 7;11(5):913. doi: 10.3390/cells11050913.
Hongge Wang et al. Evaluation of Neurofilament Light Chain as a Biomarker of Neurodegeneration in X-Linked Childhood Cerebral Adrenoleukodystrophy
- Blood Adv. 2021 Aug 24;5(16):3092-3101. doi:10.1182/bloodadvances.2020003824.
Brigitte T.A.Long-term effect of hematopoietic cell transplantation on systemic inflammation in patients with mucopolysaccharidoses
We appreciate the reviewer’s comments. We have removed the 2d-PAGE paragraph and included discussion of the PEA technique in section 2.1.4, as referred to in the previous response. The two examples of this technique applied to the study of IMDs were included in table 1 and discussed in the text as we indicated below.
In 2020 van den Broek et al.[71] investigated systemic inflammation in untreated MPS I patients and evaluated the effect of HCT (hematopoietic cell transplantation) on systemic inflammation. The authors used dried blood spot samples from patients with HCT-treated MPS using a OLINK Proseek Multiplex inflammation platform. In total, 92 markers of the OLINK inflammation panel were measured in MPS patients compared with those of age-matched control subjects. The authors observed normal leukocyte enzyme activity levels in 93% of patients post-HCT. Moreover, pretransplant samples showed clear differences between patients and controls. The protein markers that distinguished control subjects from patients pre-HCT were mainly pro-inflammatory (50%) or related to bone homeostasis and extracellular matrix degradation (33%). After 10 years of follow-up, only 5 markers (receptor activator of nuclear factor kappa-B ligand, osteoprotegerin, axis inhibition protein 1 [AXIN1], stem cell factor, and Fms-related tyrosine kinase 3 ligand) remained significantly increased, with a large fold-change difference between MPS I patients and control subjects.
In 2022 Wang et al.[79] used the OLINK Proximity Extension Assay to analyze the cerebral spinal fluid (CSF) of young males with CALD, and performed a comparative analysis using plasma samples. Using the Target 96 Neuro Exploratory panel they found that levels of 5 proteins were significantly increased in CSF. Only for neurofilament light chain (NfL) was a significant correlation observed between CSF and plasma samples. Young males with CALD showed a 11.3-fold increase in plasma NfL compared with controls. Moreover, 9 of 11 young males with CALD who underwent HCT showed a decrease in plasma NfL levels after 1 year of treatment compared with pre-HCT levels. The authors concluded that plasma NfL could constitute a useful determinant of outcomes in CALD
Minor Comments: Figure 1. The sample type should be above the two blocks "Bottom-up" and "Top down" because in the current way it is confusing.
We agree with the reviewer and have edited the figures accordingly.
Figure 4. I would specify the difference between untargeted DIA (eg SWATH) and targeted DIA (SRM/MRM and PRM).
We agree with the reviewer, we change figure 4 adding untargeted DIA for SWATH and targeted DIA for SRM/MRM and PRM. Moreover, the following text has been added to the figure legend:
Figure 4: DDA and DIA mode in proteomic analysis
In DDA mode, extracted proteins are digested and directly analyzed by single-shot DDA. The acquired data is searched against a database of known protein sequences and further processed using diverse software tools. In DIA mode two distinct forms of proteomic analysis can be performed: SWATH-MS (untargeted DIA) and SRM/MRM (targeted DIA). In SWATH-MS extracted proteins are digested and directly analyzed by single-shot analysis in order to generate the spectral library. Once the library is generated a multi-window running method is applied to individual samples: wide isolation windows span the entire MS1 m/z range and all precursor ions in the library that are found in each isolation window are fragmented in MS2. All proteins found in the library are quantified (untargeted) and all samples are processed using data analysis software to obtain the quantitative data. In MRM and SRM, the precursor ions to be analyzed are fixed (targeted) by the user, and only these are fragmented in MS2.
Figure 3 and Figure 4. When talking about targeted MS or quantitative MS the authors should mention that methods such as SRM/MRM and PRM are not simply label free, but they can be quantitative because they imply the use of an internal standard (labeled peptide), while methods based on isobaric or chemical labeling remain semiquantitative.
We agree with the reviewer: it is prudent to emphasize this important difference. The following text has been included at the end of point 2.1.3:
It should be noted that methods such as MRM/SRM/PRM-MS allow for quantification of absolute protein levels. Other previously described methods such as label-based (e.g. ITRAQ, SILAC) and non-label-based methods (e.g. SWATH) are semi quantitative methods that only indicate the fold change in protein levels between two conditions.
Targeted MS/MS allows absolute quantification of targeted proteins using isotopically labelled peptide standards (AQUA) [30–32] or protein standard absolute quantification (PSAQ) [31,33,34]. Using isotope standards simplifies sample preparation and minimizes variation between mass spectrometry devices, thereby increasing the reliability and accuracy of results.
In the review "Proteomics in Inherited Metabolic Disorders" Maria del Pilar Chantada-Vázquez and collegues describe the application of proteomics methods to the study of Inherited Metabolic Disorders (IMD).
The manuscript is well written and comprehensive, however my suggestion is to either change the title to "Mass Spectrometry (MS) in IMD" or to include in the discussion studies based on Affinity Proteomics as well as MS.
We appreciate the comment. We agree with the reviewer and have included a new subsection in section 2.1 Qualitative and Quantitative Proteomics
2.1.4 Affinity-based (probes and antibodies) proteomics approaches [35–38]
Affinity-based proteomics is a relatively new field of proteomics that seeks to characterize protein activity and protein-protein interaction. This method also allows monitoring of the functional regulation of enzymes in complex proteomes. This technology utilizes small molecule activity-based probes (APBs) or protein antibodies. The probe molecules covalently modify the enzyme active site and allow detection and affinity purification of a target enzyme population. Affinity-based antibodies are mainly generated using microarrays or directly by antibody immunoprecipitation of the whole sample. After affinity purification (pulldown based on probes or antibodies), substrates and their exact cleavage sites are identified by mass spectrometry. Moreover, cleavage sites can be quantitatively evaluated by incorporation of quantitative proteomic techniques such as iTRAQ and SILAC [39]. Thus, this technology is highly selective and can be used for analysis of complex samples such as biological fluids, cell lysates, intact cells, and even whole organisms, and can therefore be applied to the study of IMDs.
All activity-based probes share a similar structure[35,39]. They have elements required for targeting, modification, and detection of labeled proteins. Structurally, those elements can be divided into the reactive group, linker, and tag. The reactive functional group provides a covalent attachment to the catalytic residue of the enzyme active site. The linker function separates the reactive functional group from the tag. Finally, the tag enables visualization and/or purification of labeled proteins.
For the antibody microarray, the data set correlates directly with the number of antibodies included on the array and the range of their specificities [37].
Technologies that reveal the most abundant proteins found in certain samples types (e.g. serum) are crucial for the development of biomarkers that can be used as measurable indicators of disease severity and progression, patient stratification, and drug development. Proximity Extension Assay (PEA) is a novel approach based in the interaction between protein antibodies and DNA [38] that has been commercially developed for the analysis of secreted proteins in serum and blood plasma. This technology can translate protein information into actionable knowledge by linking protein-specific antibodies to DNA-encoded tags. It offers high specificity and sensitivity (sub-pg/ml), enabling high multiplex assays with coverage across a broad dynamic range (~9 log), while consuming minimal quantities of sample. In PEA, matched pairs of oligonucleotide-labeled antibodies bind to their target antigens in a pairwise manner. Upon antibody binding, the matched oligonucleotides are brought into proximity, and using a DNA polymerase a PCR target sequence is created, amplified, detected, and quantified. This downstream process analysis is usually carried out by qPCR or by techniques such as next-generation sequencing (NGS).
The above are all recent technologies, and the coming years will likely see continued growth in the development and application of affinity-based proteomic approaches. The use of probes that target diverse enzyme families has led to a range of applications for activity-based proteomics, in the same way that linking proteins to proteins has provided information about the interactome and protein-DNA interactions have provided further knowledge about cell processes. Therefore, as in other proteomics approaches, comparison of enzyme activity profiles and protein-protein or protein-DNA interactions (based on antibody precipitation) in healthy versus diseased samples can aid the identification of novel biomarkers and drug targets. The results obtained to date show that affinity-based proteomics offer great promise as imaging tools for disease diagnosis and preclinical and clinical testing of therapeutic agents in vivo.
We have also included a new study in table 1 in which the authors used affinity-based methods applied to IMDs, in this case Duchenne muscular dystrophy.
Duchenne muscular dystrophy (DMD; OMIM310200) is a rare X-linked genetic disease with an incidence rate of 1:5,000. DMD is caused by frame-disrupting mutations in the gene encoding dystrophin, resulting in loss of dystrophin [90]. Affected boys typically present clinical signs in the first few years of life, with features suggestive of muscle weakness and often with global developmental delay. Progressive muscle weakness leads to loss of ambulation by the age of 10, and if untreated, to fatal cardio respiratory insufficiency by late adolescence. Establishing a correct diagnosis in dystrophinopathies requires a multidisciplinary approach involving pediatricians, geneticists, and neurologists to define the severity of the clinical phenotype by means of genetic, enzymatic, and immunohistochemical tests [90]. The application of proteomics to the study of dystrophinopathies could facilitate both diagnosis and treatment. In 2014 Ayoglu et al. [36] explored the possibility of identifying circulating candidate protein markers in rare diseases applying an affinity-based proteomics approach to generate a proteomic profile in blood samples from muscular dystrophy patients versus controls. The authors used an antibody bead array platform with 384 antibodies. Based on the results obtained from analysis of both serum and plasma, they identified 11 proteins associated with muscular dystrophy, of which 4 were upregulated in blood from muscular dystrophy patients: carbonic anhydrase III (CA3), myosin light chain 3 (MYL3), mitochondrial malate dehydrogenase 2 (MDH2), and electron transfer flavoprotein A (ETFA). These interesting data could aid the development of new clinical tools for management of dystrophinopathies.
The general description about proteomics methods could be revised cutting (or only mentioning) 2D-PAGE which is a relatively old technique, while including methods such as high multiplexed protein profiling using Proximity Extension Assays (PEA) or Somascan.
A few examples of possibly relevant studies are:
- Cells. 2022 Mar 7;11(5):913. doi: 10.3390/cells11050913.
Hongge Wang et al. Evaluation of Neurofilament Light Chain as a Biomarker of Neurodegeneration in X-Linked Childhood Cerebral Adrenoleukodystrophy
- Blood Adv. 2021 Aug 24;5(16):3092-3101. doi:10.1182/bloodadvances.2020003824.
Brigitte T.A.Long-term effect of hematopoietic cell transplantation on systemic inflammation in patients with mucopolysaccharidoses
We appreciate the reviewer’s comments. We have removed the 2d-PAGE paragraph and included discussion of the PEA technique in section 2.1.4, as referred to in the previous response. The two examples of this technique applied to the study of IMDs were included in table 1 and discussed in the text as we indicated below.
In 2020 van den Broek et al.[71] investigated systemic inflammation in untreated MPS I patients and evaluated the effect of HCT (hematopoietic cell transplantation) on systemic inflammation. The authors used dried blood spot samples from patients with HCT-treated MPS using a OLINK Proseek Multiplex inflammation platform. In total, 92 markers of the OLINK inflammation panel were measured in MPS patients compared with those of age-matched control subjects. The authors observed normal leukocyte enzyme activity levels in 93% of patients post-HCT. Moreover, pretransplant samples showed clear differences between patients and controls. The protein markers that distinguished control subjects from patients pre-HCT were mainly pro-inflammatory (50%) or related to bone homeostasis and extracellular matrix degradation (33%). After 10 years of follow-up, only 5 markers (receptor activator of nuclear factor kappa-B ligand, osteoprotegerin, axis inhibition protein 1 [AXIN1], stem cell factor, and Fms-related tyrosine kinase 3 ligand) remained significantly increased, with a large fold-change difference between MPS I patients and control subjects.
In 2022 Wang et al.[79] used the OLINK Proximity Extension Assay to analyze the cerebral spinal fluid (CSF) of young males with CALD, and performed a comparative analysis using plasma samples. Using the Target 96 Neuro Exploratory panel they found that levels of 5 proteins were significantly increased in CSF. Only for neurofilament light chain (NfL) was a significant correlation observed between CSF and plasma samples. Young males with CALD showed a 11.3-fold increase in plasma NfL compared with controls. Moreover, 9 of 11 young males with CALD who underwent HCT showed a decrease in plasma NfL levels after 1 year of treatment compared with pre-HCT levels. The authors concluded that plasma NfL could constitute a useful determinant of outcomes in CALD
Minor Comments: Figure 1. The sample type should be above the two blocks "Bottom-up" and "Top down" because in the current way it is confusing.
We agree with the reviewer and have edited the figures accordingly.
Figure 4. I would specify the difference between untargeted DIA (eg SWATH) and targeted DIA (SRM/MRM and PRM).
We agree with the reviewer, we change figure 4 adding untargeted DIA for SWATH and targeted DIA for SRM/MRM and PRM. Moreover, the following text has been added to the figure legend:
Figure 4: DDA and DIA mode in proteomic analysis
In DDA mode, extracted proteins are digested and directly analyzed by single-shot DDA. The acquired data is searched against a database of known protein sequences and further processed using diverse software tools. In DIA mode two distinct forms of proteomic analysis can be performed: SWATH-MS (untargeted DIA) and SRM/MRM (targeted DIA). In SWATH-MS extracted proteins are digested and directly analyzed by single-shot analysis in order to generate the spectral library. Once the library is generated a multi-window running method is applied to individual samples: wide isolation windows span the entire MS1 m/z range and all precursor ions in the library that are found in each isolation window are fragmented in MS2. All proteins found in the library are quantified (untargeted) and all samples are processed using data analysis software to obtain the quantitative data. In MRM and SRM, the precursor ions to be analyzed are fixed (targeted) by the user, and only these are fragmented in MS2.
Figure 3 and Figure 4. When talking about targeted MS or quantitative MS the authors should mention that methods such as SRM/MRM and PRM are not simply label free, but they can be quantitative because they imply the use of an internal standard (labeled peptide), while methods based on isobaric or chemical labeling remain semiquantitative.
We agree with the reviewer: it is prudent to emphasize this important difference. The following text has been included at the end of point 2.1.3:
It should be noted that methods such as MRM/SRM/PRM-MS allow for quantification of absolute protein levels. Other previously described methods such as label-based (e.g. ITRAQ, SILAC) and non-label-based methods (e.g. SWATH) are semi quantitative methods that only indicate the fold change in protein levels between two conditions.
Targeted MS/MS allows absolute quantification of targeted proteins using isotopically labelled peptide standards (AQUA) [30–32] or protein standard absolute quantification (PSAQ) [31,33,34]. Using isotope standards simplifies sample preparation and minimizes variation between mass spectrometry devices, thereby increasing the reliability and accuracy of results.
In the review "Proteomics in Inherited Metabolic Disorders" Maria del Pilar Chantada-Vázquez and collegues describe the application of proteomics methods to the study of Inherited Metabolic Disorders (IMD).
The manuscript is well written and comprehensive, however my suggestion is to either change the title to "Mass Spectrometry (MS) in IMD" or to include in the discussion studies based on Affinity Proteomics as well as MS.
We appreciate the comment. We agree with the reviewer and have included a new subsection in section 2.1 Qualitative and Quantitative Proteomics
2.1.4 Affinity-based (probes and antibodies) proteomics approaches [35–38]
Affinity-based proteomics is a relatively new field of proteomics that seeks to characterize protein activity and protein-protein interaction. This method also allows monitoring of the functional regulation of enzymes in complex proteomes. This technology utilizes small molecule activity-based probes (APBs) or protein antibodies. The probe molecules covalently modify the enzyme active site and allow detection and affinity purification of a target enzyme population. Affinity-based antibodies are mainly generated using microarrays or directly by antibody immunoprecipitation of the whole sample. After affinity purification (pulldown based on probes or antibodies), substrates and their exact cleavage sites are identified by mass spectrometry. Moreover, cleavage sites can be quantitatively evaluated by incorporation of quantitative proteomic techniques such as iTRAQ and SILAC [39]. Thus, this technology is highly selective and can be used for analysis of complex samples such as biological fluids, cell lysates, intact cells, and even whole organisms, and can therefore be applied to the study of IMDs.
All activity-based probes share a similar structure[35,39]. They have elements required for targeting, modification, and detection of labeled proteins. Structurally, those elements can be divided into the reactive group, linker, and tag. The reactive functional group provides a covalent attachment to the catalytic residue of the enzyme active site. The linker function separates the reactive functional group from the tag. Finally, the tag enables visualization and/or purification of labeled proteins.
For the antibody microarray, the data set correlates directly with the number of antibodies included on the array and the range of their specificities [37].
Technologies that reveal the most abundant proteins found in certain samples types (e.g. serum) are crucial for the development of biomarkers that can be used as measurable indicators of disease severity and progression, patient stratification, and drug development. Proximity Extension Assay (PEA) is a novel approach based in the interaction between protein antibodies and DNA [38] that has been commercially developed for the analysis of secreted proteins in serum and blood plasma. This technology can translate protein information into actionable knowledge by linking protein-specific antibodies to DNA-encoded tags. It offers high specificity and sensitivity (sub-pg/ml), enabling high multiplex assays with coverage across a broad dynamic range (~9 log), while consuming minimal quantities of sample. In PEA, matched pairs of oligonucleotide-labeled antibodies bind to their target antigens in a pairwise manner. Upon antibody binding, the matched oligonucleotides are brought into proximity, and using a DNA polymerase a PCR target sequence is created, amplified, detected, and quantified. This downstream process analysis is usually carried out by qPCR or by techniques such as next-generation sequencing (NGS).
The above are all recent technologies, and the coming years will likely see continued growth in the development and application of affinity-based proteomic approaches. The use of probes that target diverse enzyme families has led to a range of applications for activity-based proteomics, in the same way that linking proteins to proteins has provided information about the interactome and protein-DNA interactions have provided further knowledge about cell processes. Therefore, as in other proteomics approaches, comparison of enzyme activity profiles and protein-protein or protein-DNA interactions (based on antibody precipitation) in healthy versus diseased samples can aid the identification of novel biomarkers and drug targets. The results obtained to date show that affinity-based proteomics offer great promise as imaging tools for disease diagnosis and preclinical and clinical testing of therapeutic agents in vivo.
We have also included a new study in table 1 in which the authors used affinity-based methods applied to IMDs, in this case Duchenne muscular dystrophy.
Duchenne muscular dystrophy (DMD; OMIM310200) is a rare X-linked genetic disease with an incidence rate of 1:5,000. DMD is caused by frame-disrupting mutations in the gene encoding dystrophin, resulting in loss of dystrophin [90]. Affected boys typically present clinical signs in the first few years of life, with features suggestive of muscle weakness and often with global developmental delay. Progressive muscle weakness leads to loss of ambulation by the age of 10, and if untreated, to fatal cardio respiratory insufficiency by late adolescence. Establishing a correct diagnosis in dystrophinopathies requires a multidisciplinary approach involving pediatricians, geneticists, and neurologists to define the severity of the clinical phenotype by means of genetic, enzymatic, and immunohistochemical tests [90]. The application of proteomics to the study of dystrophinopathies could facilitate both diagnosis and treatment. In 2014 Ayoglu et al. [36] explored the possibility of identifying circulating candidate protein markers in rare diseases applying an affinity-based proteomics approach to generate a proteomic profile in blood samples from muscular dystrophy patients versus controls. The authors used an antibody bead array platform with 384 antibodies. Based on the results obtained from analysis of both serum and plasma, they identified 11 proteins associated with muscular dystrophy, of which 4 were upregulated in blood from muscular dystrophy patients: carbonic anhydrase III (CA3), myosin light chain 3 (MYL3), mitochondrial malate dehydrogenase 2 (MDH2), and electron transfer flavoprotein A (ETFA). These interesting data could aid the development of new clinical tools for management of dystrophinopathies.
The general description about proteomics methods could be revised cutting (or only mentioning) 2D-PAGE which is a relatively old technique, while including methods such as high multiplexed protein profiling using Proximity Extension Assays (PEA) or Somascan.
A few examples of possibly relevant studies are:
- Cells. 2022 Mar 7;11(5):913. doi: 10.3390/cells11050913.
Hongge Wang et al. Evaluation of Neurofilament Light Chain as a Biomarker of Neurodegeneration in X-Linked Childhood Cerebral Adrenoleukodystrophy
- Blood Adv. 2021 Aug 24;5(16):3092-3101. doi:10.1182/bloodadvances.2020003824.
Brigitte T.A.Long-term effect of hematopoietic cell transplantation on systemic inflammation in patients with mucopolysaccharidoses
We appreciate the reviewer’s comments. We have removed the 2d-PAGE paragraph and included discussion of the PEA technique in section 2.1.4, as referred to in the previous response. The two examples of this technique applied to the study of IMDs were included in table 1 and discussed in the text as we indicated below.
In 2020 van den Broek et al.[71] investigated systemic inflammation in untreated MPS I patients and evaluated the effect of HCT (hematopoietic cell transplantation) on systemic inflammation. The authors used dried blood spot samples from patients with HCT-treated MPS using a OLINK Proseek Multiplex inflammation platform. In total, 92 markers of the OLINK inflammation panel were measured in MPS patients compared with those of age-matched control subjects. The authors observed normal leukocyte enzyme activity levels in 93% of patients post-HCT. Moreover, pretransplant samples showed clear differences between patients and controls. The protein markers that distinguished control subjects from patients pre-HCT were mainly pro-inflammatory (50%) or related to bone homeostasis and extracellular matrix degradation (33%). After 10 years of follow-up, only 5 markers (receptor activator of nuclear factor kappa-B ligand, osteoprotegerin, axis inhibition protein 1 [AXIN1], stem cell factor, and Fms-related tyrosine kinase 3 ligand) remained significantly increased, with a large fold-change difference between MPS I patients and control subjects.
In 2022 Wang et al.[79] used the OLINK Proximity Extension Assay to analyze the cerebral spinal fluid (CSF) of young males with CALD, and performed a comparative analysis using plasma samples. Using the Target 96 Neuro Exploratory panel they found that levels of 5 proteins were significantly increased in CSF. Only for neurofilament light chain (NfL) was a significant correlation observed between CSF and plasma samples. Young males with CALD showed a 11.3-fold increase in plasma NfL compared with controls. Moreover, 9 of 11 young males with CALD who underwent HCT showed a decrease in plasma NfL levels after 1 year of treatment compared with pre-HCT levels. The authors concluded that plasma NfL could constitute a useful determinant of outcomes in CALD
Minor Comments: Figure 1. The sample type should be above the two blocks "Bottom-up" and "Top down" because in the current way it is confusing.
We agree with the reviewer and have edited the figures accordingly.
Figure 4. I would specify the difference between untargeted DIA (eg SWATH) and targeted DIA (SRM/MRM and PRM).
We agree with the reviewer, we change figure 4 adding untargeted DIA for SWATH and targeted DIA for SRM/MRM and PRM. Moreover, the following text has been added to the figure legend:
Figure 4: DDA and DIA mode in proteomic analysis
In DDA mode, extracted proteins are digested and directly analyzed by single-shot DDA. The acquired data is searched against a database of known protein sequences and further processed using diverse software tools. In DIA mode two distinct forms of proteomic analysis can be performed: SWATH-MS (untargeted DIA) and SRM/MRM (targeted DIA). In SWATH-MS extracted proteins are digested and directly analyzed by single-shot analysis in order to generate the spectral library. Once the library is generated a multi-window running method is applied to individual samples: wide isolation windows span the entire MS1 m/z range and all precursor ions in the library that are found in each isolation window are fragmented in MS2. All proteins found in the library are quantified (untargeted) and all samples are processed using data analysis software to obtain the quantitative data. In MRM and SRM, the precursor ions to be analyzed are fixed (targeted) by the user, and only these are fragmented in MS2.
Figure 3 and Figure 4. When talking about targeted MS or quantitative MS the authors should mention that methods such as SRM/MRM and PRM are not simply label free, but they can be quantitative because they imply the use of an internal standard (labeled peptide), while methods based on isobaric or chemical labeling remain semiquantitative.
We agree with the reviewer: it is prudent to emphasize this important difference. The following text has been included at the end of point 2.1.3:
It should be noted that methods such as MRM/SRM/PRM-MS allow for quantification of absolute protein levels. Other previously described methods such as label-based (e.g. ITRAQ, SILAC) and non-label-based methods (e.g. SWATH) are semi quantitative methods that only indicate the fold change in protein levels between two conditions.
Targeted MS/MS allows absolute quantification of targeted proteins using isotopically labelled peptide standards (AQUA) [30–32] or protein standard absolute quantification (PSAQ) [31,33,34]. Using isotope standards simplifies sample preparation and minimizes variation between mass spectrometry devices, thereby increasing the reliability and accuracy of results.
In the review "Proteomics in Inherited Metabolic Disorders" Maria del Pilar Chantada-Vázquez and collegues describe the application of proteomics methods to the study of Inherited Metabolic Disorders (IMD).
The manuscript is well written and comprehensive, however my suggestion is to either change the title to "Mass Spectrometry (MS) in IMD" or to include in the discussion studies based on Affinity Proteomics as well as MS.
We appreciate the comment. We agree with the reviewer and have included a new subsection in section 2.1 Qualitative and Quantitative Proteomics
2.1.4 Affinity-based (probes and antibodies) proteomics approaches [35–38]
Affinity-based proteomics is a relatively new field of proteomics that seeks to characterize protein activity and protein-protein interaction. This method also allows monitoring of the functional regulation of enzymes in complex proteomes. This technology utilizes small molecule activity-based probes (APBs) or protein antibodies. The probe molecules covalently modify the enzyme active site and allow detection and affinity purification of a target enzyme population. Affinity-based antibodies are mainly generated using microarrays or directly by antibody immunoprecipitation of the whole sample. After affinity purification (pulldown based on probes or antibodies), substrates and their exact cleavage sites are identified by mass spectrometry. Moreover, cleavage sites can be quantitatively evaluated by incorporation of quantitative proteomic techniques such as iTRAQ and SILAC [39]. Thus, this technology is highly selective and can be used for analysis of complex samples such as biological fluids, cell lysates, intact cells, and even whole organisms, and can therefore be applied to the study of IMDs.
All activity-based probes share a similar structure[35,39]. They have elements required for targeting, modification, and detection of labeled proteins. Structurally, those elements can be divided into the reactive group, linker, and tag. The reactive functional group provides a covalent attachment to the catalytic residue of the enzyme active site. The linker function separates the reactive functional group from the tag. Finally, the tag enables visualization and/or purification of labeled proteins.
For the antibody microarray, the data set correlates directly with the number of antibodies included on the array and the range of their specificities [37].
Technologies that reveal the most abundant proteins found in certain samples types (e.g. serum) are crucial for the development of biomarkers that can be used as measurable indicators of disease severity and progression, patient stratification, and drug development. Proximity Extension Assay (PEA) is a novel approach based in the interaction between protein antibodies and DNA [38] that has been commercially developed for the analysis of secreted proteins in serum and blood plasma. This technology can translate protein information into actionable knowledge by linking protein-specific antibodies to DNA-encoded tags. It offers high specificity and sensitivity (sub-pg/ml), enabling high multiplex assays with coverage across a broad dynamic range (~9 log), while consuming minimal quantities of sample. In PEA, matched pairs of oligonucleotide-labeled antibodies bind to their target antigens in a pairwise manner. Upon antibody binding, the matched oligonucleotides are brought into proximity, and using a DNA polymerase a PCR target sequence is created, amplified, detected, and quantified. This downstream process analysis is usually carried out by qPCR or by techniques such as next-generation sequencing (NGS).
The above are all recent technologies, and the coming years will likely see continued growth in the development and application of affinity-based proteomic approaches. The use of probes that target diverse enzyme families has led to a range of applications for activity-based proteomics, in the same way that linking proteins to proteins has provided information about the interactome and protein-DNA interactions have provided further knowledge about cell processes. Therefore, as in other proteomics approaches, comparison of enzyme activity profiles and protein-protein or protein-DNA interactions (based on antibody precipitation) in healthy versus diseased samples can aid the identification of novel biomarkers and drug targets. The results obtained to date show that affinity-based proteomics offer great promise as imaging tools for disease diagnosis and preclinical and clinical testing of therapeutic agents in vivo.
We have also included a new study in table 1 in which the authors used affinity-based methods applied to IMDs, in this case Duchenne muscular dystrophy.
Duchenne muscular dystrophy (DMD; OMIM310200) is a rare X-linked genetic disease with an incidence rate of 1:5,000. DMD is caused by frame-disrupting mutations in the gene encoding dystrophin, resulting in loss of dystrophin [90]. Affected boys typically present clinical signs in the first few years of life, with features suggestive of muscle weakness and often with global developmental delay. Progressive muscle weakness leads to loss of ambulation by the age of 10, and if untreated, to fatal cardio respiratory insufficiency by late adolescence. Establishing a correct diagnosis in dystrophinopathies requires a multidisciplinary approach involving pediatricians, geneticists, and neurologists to define the severity of the clinical phenotype by means of genetic, enzymatic, and immunohistochemical tests [90]. The application of proteomics to the study of dystrophinopathies could facilitate both diagnosis and treatment. In 2014 Ayoglu et al. [36] explored the possibility of identifying circulating candidate protein markers in rare diseases applying an affinity-based proteomics approach to generate a proteomic profile in blood samples from muscular dystrophy patients versus controls. The authors used an antibody bead array platform with 384 antibodies. Based on the results obtained from analysis of both serum and plasma, they identified 11 proteins associated with muscular dystrophy, of which 4 were upregulated in blood from muscular dystrophy patients: carbonic anhydrase III (CA3), myosin light chain 3 (MYL3), mitochondrial malate dehydrogenase 2 (MDH2), and electron transfer flavoprotein A (ETFA). These interesting data could aid the development of new clinical tools for management of dystrophinopathies.
The general description about proteomics methods could be revised cutting (or only mentioning) 2D-PAGE which is a relatively old technique, while including methods such as high multiplexed protein profiling using Proximity Extension Assays (PEA) or Somascan.
A few examples of possibly relevant studies are:
- Cells. 2022 Mar 7;11(5):913. doi: 10.3390/cells11050913.
Hongge Wang et al. Evaluation of Neurofilament Light Chain as a Biomarker of Neurodegeneration in X-Linked Childhood Cerebral Adrenoleukodystrophy
- Blood Adv. 2021 Aug 24;5(16):3092-3101. doi:10.1182/bloodadvances.2020003824.
Brigitte T.A.Long-term effect of hematopoietic cell transplantation on systemic inflammation in patients with mucopolysaccharidoses
We appreciate the reviewer’s comments. We have removed the 2d-PAGE paragraph and included discussion of the PEA technique in section 2.1.4, as referred to in the previous response. The two examples of this technique applied to the study of IMDs were included in table 1 and discussed in the text as we indicated below.
In 2020 van den Broek et al.[71] investigated systemic inflammation in untreated MPS I patients and evaluated the effect of HCT (hematopoietic cell transplantation) on systemic inflammation. The authors used dried blood spot samples from patients with HCT-treated MPS using a OLINK Proseek Multiplex inflammation platform. In total, 92 markers of the OLINK inflammation panel were measured in MPS patients compared with those of age-matched control subjects. The authors observed normal leukocyte enzyme activity levels in 93% of patients post-HCT. Moreover, pretransplant samples showed clear differences between patients and controls. The protein markers that distinguished control subjects from patients pre-HCT were mainly pro-inflammatory (50%) or related to bone homeostasis and extracellular matrix degradation (33%). After 10 years of follow-up, only 5 markers (receptor activator of nuclear factor kappa-B ligand, osteoprotegerin, axis inhibition protein 1 [AXIN1], stem cell factor, and Fms-related tyrosine kinase 3 ligand) remained significantly increased, with a large fold-change difference between MPS I patients and control subjects.
In 2022 Wang et al.[79] used the OLINK Proximity Extension Assay to analyze the cerebral spinal fluid (CSF) of young males with CALD, and performed a comparative analysis using plasma samples. Using the Target 96 Neuro Exploratory panel they found that levels of 5 proteins were significantly increased in CSF. Only for neurofilament light chain (NfL) was a significant correlation observed between CSF and plasma samples. Young males with CALD showed a 11.3-fold increase in plasma NfL compared with controls. Moreover, 9 of 11 young males with CALD who underwent HCT showed a decrease in plasma NfL levels after 1 year of treatment compared with pre-HCT levels. The authors concluded that plasma NfL could constitute a useful determinant of outcomes in CALD
Minor Comments: Figure 1. The sample type should be above the two blocks "Bottom-up" and "Top down" because in the current way it is confusing.
We agree with the reviewer and have edited the figures accordingly.
Figure 4. I would specify the difference between untargeted DIA (eg SWATH) and targeted DIA (SRM/MRM and PRM).
We agree with the reviewer, we change figure 4 adding untargeted DIA for SWATH and targeted DIA for SRM/MRM and PRM. Moreover, the following text has been added to the figure legend:
Figure 4: DDA and DIA mode in proteomic analysis
In DDA mode, extracted proteins are digested and directly analyzed by single-shot DDA. The acquired data is searched against a database of known protein sequences and further processed using diverse software tools. In DIA mode two distinct forms of proteomic analysis can be performed: SWATH-MS (untargeted DIA) and SRM/MRM (targeted DIA). In SWATH-MS extracted proteins are digested and directly analyzed by single-shot analysis in order to generate the spectral library. Once the library is generated a multi-window running method is applied to individual samples: wide isolation windows span the entire MS1 m/z range and all precursor ions in the library that are found in each isolation window are fragmented in MS2. All proteins found in the library are quantified (untargeted) and all samples are processed using data analysis software to obtain the quantitative data. In MRM and SRM, the precursor ions to be analyzed are fixed (targeted) by the user, and only these are fragmented in MS2.
Figure 3 and Figure 4. When talking about targeted MS or quantitative MS the authors should mention that methods such as SRM/MRM and PRM are not simply label free, but they can be quantitative because they imply the use of an internal standard (labeled peptide), while methods based on isobaric or chemical labeling remain semiquantitative.
We agree with the reviewer: it is prudent to emphasize this important difference. The following text has been included at the end of point 2.1.3:
It should be noted that methods such as MRM/SRM/PRM-MS allow for quantification of absolute protein levels. Other previously described methods such as label-based (e.g. ITRAQ, SILAC) and non-label-based methods (e.g. SWATH) are semi quantitative methods that only indicate the fold change in protein levels between two conditions.
Targeted MS/MS allows absolute quantification of targeted proteins using isotopically labelled peptide standards (AQUA) [30–32] or protein standard absolute quantification (PSAQ) [31,33,34]. Using isotope standards simplifies sample preparation and minimizes variation between mass spectrometry devices, thereby increasing the reliability and accuracy of results.
In the review "Proteomics in Inherited Metabolic Disorders" Maria del Pilar Chantada-Vázquez and collegues describe the application of proteomics methods to the study of Inherited Metabolic Disorders (IMD).
The manuscript is well written and comprehensive, however my suggestion is to either change the title to "Mass Spectrometry (MS) in IMD" or to include in the discussion studies based on Affinity Proteomics as well as MS.
We appreciate the comment. We agree with the reviewer and have included a new subsection in section 2.1 Qualitative and Quantitative Proteomics
2.1.4 Affinity-based (probes and antibodies) proteomics approaches [35–38]
Affinity-based proteomics is a relatively new field of proteomics that seeks to characterize protein activity and protein-protein interaction. This method also allows monitoring of the functional regulation of enzymes in complex proteomes. This technology utilizes small molecule activity-based probes (APBs) or protein antibodies. The probe molecules covalently modify the enzyme active site and allow detection and affinity purification of a target enzyme population. Affinity-based antibodies are mainly generated using microarrays or directly by antibody immunoprecipitation of the whole sample. After affinity purification (pulldown based on probes or antibodies), substrates and their exact cleavage sites are identified by mass spectrometry. Moreover, cleavage sites can be quantitatively evaluated by incorporation of quantitative proteomic techniques such as iTRAQ and SILAC [39]. Thus, this technology is highly selective and can be used for analysis of complex samples such as biological fluids, cell lysates, intact cells, and even whole organisms, and can therefore be applied to the study of IMDs.
All activity-based probes share a similar structure[35,39]. They have elements required for targeting, modification, and detection of labeled proteins. Structurally, those elements can be divided into the reactive group, linker, and tag. The reactive functional group provides a covalent attachment to the catalytic residue of the enzyme active site. The linker function separates the reactive functional group from the tag. Finally, the tag enables visualization and/or purification of labeled proteins.
For the antibody microarray, the data set correlates directly with the number of antibodies included on the array and the range of their specificities [37].
Technologies that reveal the most abundant proteins found in certain samples types (e.g. serum) are crucial for the development of biomarkers that can be used as measurable indicators of disease severity and progression, patient stratification, and drug development. Proximity Extension Assay (PEA) is a novel approach based in the interaction between protein antibodies and DNA [38] that has been commercially developed for the analysis of secreted proteins in serum and blood plasma. This technology can translate protein information into actionable knowledge by linking protein-specific antibodies to DNA-encoded tags. It offers high specificity and sensitivity (sub-pg/ml), enabling high multiplex assays with coverage across a broad dynamic range (~9 log), while consuming minimal quantities of sample. In PEA, matched pairs of oligonucleotide-labeled antibodies bind to their target antigens in a pairwise manner. Upon antibody binding, the matched oligonucleotides are brought into proximity, and using a DNA polymerase a PCR target sequence is created, amplified, detected, and quantified. This downstream process analysis is usually carried out by qPCR or by techniques such as next-generation sequencing (NGS).
The above are all recent technologies, and the coming years will likely see continued growth in the development and application of affinity-based proteomic approaches. The use of probes that target diverse enzyme families has led to a range of applications for activity-based proteomics, in the same way that linking proteins to proteins has provided information about the interactome and protein-DNA interactions have provided further knowledge about cell processes. Therefore, as in other proteomics approaches, comparison of enzyme activity profiles and protein-protein or protein-DNA interactions (based on antibody precipitation) in healthy versus diseased samples can aid the identification of novel biomarkers and drug targets. The results obtained to date show that affinity-based proteomics offer great promise as imaging tools for disease diagnosis and preclinical and clinical testing of therapeutic agents in vivo.
We have also included a new study in table 1 in which the authors used affinity-based methods applied to IMDs, in this case Duchenne muscular dystrophy.
Duchenne muscular dystrophy (DMD; OMIM310200) is a rare X-linked genetic disease with an incidence rate of 1:5,000. DMD is caused by frame-disrupting mutations in the gene encoding dystrophin, resulting in loss of dystrophin [90]. Affected boys typically present clinical signs in the first few years of life, with features suggestive of muscle weakness and often with global developmental delay. Progressive muscle weakness leads to loss of ambulation by the age of 10, and if untreated, to fatal cardio respiratory insufficiency by late adolescence. Establishing a correct diagnosis in dystrophinopathies requires a multidisciplinary approach involving pediatricians, geneticists, and neurologists to define the severity of the clinical phenotype by means of genetic, enzymatic, and immunohistochemical tests [90]. The application of proteomics to the study of dystrophinopathies could facilitate both diagnosis and treatment. In 2014 Ayoglu et al. [36] explored the possibility of identifying circulating candidate protein markers in rare diseases applying an affinity-based proteomics approach to generate a proteomic profile in blood samples from muscular dystrophy patients versus controls. The authors used an antibody bead array platform with 384 antibodies. Based on the results obtained from analysis of both serum and plasma, they identified 11 proteins associated with muscular dystrophy, of which 4 were upregulated in blood from muscular dystrophy patients: carbonic anhydrase III (CA3), myosin light chain 3 (MYL3), mitochondrial malate dehydrogenase 2 (MDH2), and electron transfer flavoprotein A (ETFA). These interesting data could aid the development of new clinical tools for management of dystrophinopathies.
The general description about proteomics methods could be revised cutting (or only mentioning) 2D-PAGE which is a relatively old technique, while including methods such as high multiplexed protein profiling using Proximity Extension Assays (PEA) or Somascan.
A few examples of possibly relevant studies are:
- Cells. 2022 Mar 7;11(5):913. doi: 10.3390/cells11050913.
Hongge Wang et al. Evaluation of Neurofilament Light Chain as a Biomarker of Neurodegeneration in X-Linked Childhood Cerebral Adrenoleukodystrophy
- Blood Adv. 2021 Aug 24;5(16):3092-3101. doi:10.1182/bloodadvances.2020003824.
Brigitte T.A.Long-term effect of hematopoietic cell transplantation on systemic inflammation in patients with mucopolysaccharidoses
We appreciate the reviewer’s comments. We have removed the 2d-PAGE paragraph and included discussion of the PEA technique in section 2.1.4, as referred to in the previous response. The two examples of this technique applied to the study of IMDs were included in table 1 and discussed in the text as we indicated below.
In 2020 van den Broek et al.[71] investigated systemic inflammation in untreated MPS I patients and evaluated the effect of HCT (hematopoietic cell transplantation) on systemic inflammation. The authors used dried blood spot samples from patients with HCT-treated MPS using a OLINK Proseek Multiplex inflammation platform. In total, 92 markers of the OLINK inflammation panel were measured in MPS patients compared with those of age-matched control subjects. The authors observed normal leukocyte enzyme activity levels in 93% of patients post-HCT. Moreover, pretransplant samples showed clear differences between patients and controls. The protein markers that distinguished control subjects from patients pre-HCT were mainly pro-inflammatory (50%) or related to bone homeostasis and extracellular matrix degradation (33%). After 10 years of follow-up, only 5 markers (receptor activator of nuclear factor kappa-B ligand, osteoprotegerin, axis inhibition protein 1 [AXIN1], stem cell factor, and Fms-related tyrosine kinase 3 ligand) remained significantly increased, with a large fold-change difference between MPS I patients and control subjects.
In 2022 Wang et al.[79] used the OLINK Proximity Extension Assay to analyze the cerebral spinal fluid (CSF) of young males with CALD, and performed a comparative analysis using plasma samples. Using the Target 96 Neuro Exploratory panel they found that levels of 5 proteins were significantly increased in CSF. Only for neurofilament light chain (NfL) was a significant correlation observed between CSF and plasma samples. Young males with CALD showed a 11.3-fold increase in plasma NfL compared with controls. Moreover, 9 of 11 young males with CALD who underwent HCT showed a decrease in plasma NfL levels after 1 year of treatment compared with pre-HCT levels. The authors concluded that plasma NfL could constitute a useful determinant of outcomes in CALD
Minor Comments: Figure 1. The sample type should be above the two blocks "Bottom-up" and "Top down" because in the current way it is confusing.
We agree with the reviewer and have edited the figures accordingly.
Figure 4. I would specify the difference between untargeted DIA (eg SWATH) and targeted DIA (SRM/MRM and PRM).
We agree with the reviewer, we change figure 4 adding untargeted DIA for SWATH and targeted DIA for SRM/MRM and PRM. Moreover, the following text has been added to the figure legend:
Figure 4: DDA and DIA mode in proteomic analysis
In DDA mode, extracted proteins are digested and directly analyzed by single-shot DDA. The acquired data is searched against a database of known protein sequences and further processed using diverse software tools. In DIA mode two distinct forms of proteomic analysis can be performed: SWATH-MS (untargeted DIA) and SRM/MRM (targeted DIA). In SWATH-MS extracted proteins are digested and directly analyzed by single-shot analysis in order to generate the spectral library. Once the library is generated a multi-window running method is applied to individual samples: wide isolation windows span the entire MS1 m/z range and all precursor ions in the library that are found in each isolation window are fragmented in MS2. All proteins found in the library are quantified (untargeted) and all samples are processed using data analysis software to obtain the quantitative data. In MRM and SRM, the precursor ions to be analyzed are fixed (targeted) by the user, and only these are fragmented in MS2.
Figure 3 and Figure 4. When talking about targeted MS or quantitative MS the authors should mention that methods such as SRM/MRM and PRM are not simply label free, but they can be quantitative because they imply the use of an internal standard (labeled peptide), while methods based on isobaric or chemical labeling remain semiquantitative.
We agree with the reviewer: it is prudent to emphasize this important difference. The following text has been included at the end of point 2.1.3:
It should be noted that methods such as MRM/SRM/PRM-MS allow for quantification of absolute protein levels. Other previously described methods such as label-based (e.g. ITRAQ, SILAC) and non-label-based methods (e.g. SWATH) are semi quantitative methods that only indicate the fold change in protein levels between two conditions.
Targeted MS/MS allows absolute quantification of targeted proteins using isotopically labelled peptide standards (AQUA) [30–32] or protein standard absolute quantification (PSAQ) [31,33,34]. Using isotope standards simplifies sample preparation and minimizes variation between mass spectrometry devices, thereby increasing the reliability and accuracy of results.
In the review "Proteomics in Inherited Metabolic Disorders" Maria del Pilar Chantada-Vázquez and collegues describe the application of proteomics methods to the study of Inherited Metabolic Disorders (IMD).
The manuscript is well written and comprehensive, however my suggestion is to either change the title to "Mass Spectrometry (MS) in IMD" or to include in the discussion studies based on Affinity Proteomics as well as MS.
We appreciate the comment. We agree with the reviewer and have included a new subsection in section 2.1 Qualitative and Quantitative Proteomics
2.1.4 Affinity-based (probes and antibodies) proteomics approaches [35–38]
Affinity-based proteomics is a relatively new field of proteomics that seeks to characterize protein activity and protein-protein interaction. This method also allows monitoring of the functional regulation of enzymes in complex proteomes. This technology utilizes small molecule activity-based probes (APBs) or protein antibodies. The probe molecules covalently modify the enzyme active site and allow detection and affinity purification of a target enzyme population. Affinity-based antibodies are mainly generated using microarrays or directly by antibody immunoprecipitation of the whole sample. After affinity purification (pulldown based on probes or antibodies), substrates and their exact cleavage sites are identified by mass spectrometry. Moreover, cleavage sites can be quantitatively evaluated by incorporation of quantitative proteomic techniques such as iTRAQ and SILAC [39]. Thus, this technology is highly selective and can be used for analysis of complex samples such as biological fluids, cell lysates, intact cells, and even whole organisms, and can therefore be applied to the study of IMDs.
All activity-based probes share a similar structure[35,39]. They have elements required for targeting, modification, and detection of labeled proteins. Structurally, those elements can be divided into the reactive group, linker, and tag. The reactive functional group provides a covalent attachment to the catalytic residue of the enzyme active site. The linker function separates the reactive functional group from the tag. Finally, the tag enables visualization and/or purification of labeled proteins.
For the antibody microarray, the data set correlates directly with the number of antibodies included on the array and the range of their specificities [37].
Technologies that reveal the most abundant proteins found in certain samples types (e.g. serum) are crucial for the development of biomarkers that can be used as measurable indicators of disease severity and progression, patient stratification, and drug development. Proximity Extension Assay (PEA) is a novel approach based in the interaction between protein antibodies and DNA [38] that has been commercially developed for the analysis of secreted proteins in serum and blood plasma. This technology can translate protein information into actionable knowledge by linking protein-specific antibodies to DNA-encoded tags. It offers high specificity and sensitivity (sub-pg/ml), enabling high multiplex assays with coverage across a broad dynamic range (~9 log), while consuming minimal quantities of sample. In PEA, matched pairs of oligonucleotide-labeled antibodies bind to their target antigens in a pairwise manner. Upon antibody binding, the matched oligonucleotides are brought into proximity, and using a DNA polymerase a PCR target sequence is created, amplified, detected, and quantified. This downstream process analysis is usually carried out by qPCR or by techniques such as next-generation sequencing (NGS).
The above are all recent technologies, and the coming years will likely see continued growth in the development and application of affinity-based proteomic approaches. The use of probes that target diverse enzyme families has led to a range of applications for activity-based proteomics, in the same way that linking proteins to proteins has provided information about the interactome and protein-DNA interactions have provided further knowledge about cell processes. Therefore, as in other proteomics approaches, comparison of enzyme activity profiles and protein-protein or protein-DNA interactions (based on antibody precipitation) in healthy versus diseased samples can aid the identification of novel biomarkers and drug targets. The results obtained to date show that affinity-based proteomics offer great promise as imaging tools for disease diagnosis and preclinical and clinical testing of therapeutic agents in vivo.
We have also included a new study in table 1 in which the authors used affinity-based methods applied to IMDs, in this case Duchenne muscular dystrophy.
Duchenne muscular dystrophy (DMD; OMIM310200) is a rare X-linked genetic disease with an incidence rate of 1:5,000. DMD is caused by frame-disrupting mutations in the gene encoding dystrophin, resulting in loss of dystrophin [90]. Affected boys typically present clinical signs in the first few years of life, with features suggestive of muscle weakness and often with global developmental delay. Progressive muscle weakness leads to loss of ambulation by the age of 10, and if untreated, to fatal cardio respiratory insufficiency by late adolescence. Establishing a correct diagnosis in dystrophinopathies requires a multidisciplinary approach involving pediatricians, geneticists, and neurologists to define the severity of the clinical phenotype by means of genetic, enzymatic, and immunohistochemical tests [90]. The application of proteomics to the study of dystrophinopathies could facilitate both diagnosis and treatment. In 2014 Ayoglu et al. [36] explored the possibility of identifying circulating candidate protein markers in rare diseases applying an affinity-based proteomics approach to generate a proteomic profile in blood samples from muscular dystrophy patients versus controls. The authors used an antibody bead array platform with 384 antibodies. Based on the results obtained from analysis of both serum and plasma, they identified 11 proteins associated with muscular dystrophy, of which 4 were upregulated in blood from muscular dystrophy patients: carbonic anhydrase III (CA3), myosin light chain 3 (MYL3), mitochondrial malate dehydrogenase 2 (MDH2), and electron transfer flavoprotein A (ETFA). These interesting data could aid the development of new clinical tools for management of dystrophinopathies.
The general description about proteomics methods could be revised cutting (or only mentioning) 2D-PAGE which is a relatively old technique, while including methods such as high multiplexed protein profiling using Proximity Extension Assays (PEA) or Somascan.
A few examples of possibly relevant studies are:
- Cells. 2022 Mar 7;11(5):913. doi: 10.3390/cells11050913.
Hongge Wang et al. Evaluation of Neurofilament Light Chain as a Biomarker of Neurodegeneration in X-Linked Childhood Cerebral Adrenoleukodystrophy
- Blood Adv. 2021 Aug 24;5(16):3092-3101. doi:10.1182/bloodadvances.2020003824.
Brigitte T.A.Long-term effect of hematopoietic cell transplantation on systemic inflammation in patients with mucopolysaccharidoses
We appreciate the reviewer’s comments. We have removed the 2d-PAGE paragraph and included discussion of the PEA technique in section 2.1.4, as referred to in the previous response. The two examples of this technique applied to the study of IMDs were included in table 1 and discussed in the text as we indicated below.
In 2020 van den Broek et al.[71] investigated systemic inflammation in untreated MPS I patients and evaluated the effect of HCT (hematopoietic cell transplantation) on systemic inflammation. The authors used dried blood spot samples from patients with HCT-treated MPS using a OLINK Proseek Multiplex inflammation platform. In total, 92 markers of the OLINK inflammation panel were measured in MPS patients compared with those of age-matched control subjects. The authors observed normal leukocyte enzyme activity levels in 93% of patients post-HCT. Moreover, pretransplant samples showed clear differences between patients and controls. The protein markers that distinguished control subjects from patients pre-HCT were mainly pro-inflammatory (50%) or related to bone homeostasis and extracellular matrix degradation (33%). After 10 years of follow-up, only 5 markers (receptor activator of nuclear factor kappa-B ligand, osteoprotegerin, axis inhibition protein 1 [AXIN1], stem cell factor, and Fms-related tyrosine kinase 3 ligand) remained significantly increased, with a large fold-change difference between MPS I patients and control subjects.
In 2022 Wang et al.[79] used the OLINK Proximity Extension Assay to analyze the cerebral spinal fluid (CSF) of young males with CALD, and performed a comparative analysis using plasma samples. Using the Target 96 Neuro Exploratory panel they found that levels of 5 proteins were significantly increased in CSF. Only for neurofilament light chain (NfL) was a significant correlation observed between CSF and plasma samples. Young males with CALD showed a 11.3-fold increase in plasma NfL compared with controls. Moreover, 9 of 11 young males with CALD who underwent HCT showed a decrease in plasma NfL levels after 1 year of treatment compared with pre-HCT levels. The authors concluded that plasma NfL could constitute a useful determinant of outcomes in CALD
Minor Comments: Figure 1. The sample type should be above the two blocks "Bottom-up" and "Top down" because in the current way it is confusing.
We agree with the reviewer and have edited the figures accordingly.
Figure 4. I would specify the difference between untargeted DIA (eg SWATH) and targeted DIA (SRM/MRM and PRM).
We agree with the reviewer, we change figure 4 adding untargeted DIA for SWATH and targeted DIA for SRM/MRM and PRM. Moreover, the following text has been added to the figure legend:
Figure 4: DDA and DIA mode in proteomic analysis
In DDA mode, extracted proteins are digested and directly analyzed by single-shot DDA. The acquired data is searched against a database of known protein sequences and further processed using diverse software tools. In DIA mode two distinct forms of proteomic analysis can be performed: SWATH-MS (untargeted DIA) and SRM/MRM (targeted DIA). In SWATH-MS extracted proteins are digested and directly analyzed by single-shot analysis in order to generate the spectral library. Once the library is generated a multi-window running method is applied to individual samples: wide isolation windows span the entire MS1 m/z range and all precursor ions in the library that are found in each isolation window are fragmented in MS2. All proteins found in the library are quantified (untargeted) and all samples are processed using data analysis software to obtain the quantitative data. In MRM and SRM, the precursor ions to be analyzed are fixed (targeted) by the user, and only these are fragmented in MS2.
Figure 3 and Figure 4. When talking about targeted MS or quantitative MS the authors should mention that methods such as SRM/MRM and PRM are not simply label free, but they can be quantitative because they imply the use of an internal standard (labeled peptide), while methods based on isobaric or chemical labeling remain semiquantitative.
We agree with the reviewer: it is prudent to emphasize this important difference. The following text has been included at the end of point 2.1.3:
It should be noted that methods such as MRM/SRM/PRM-MS allow for quantification of absolute protein levels. Other previously described methods such as label-based (e.g. ITRAQ, SILAC) and non-label-based methods (e.g. SWATH) are semi quantitative methods that only indicate the fold change in protein levels between two conditions.
Targeted MS/MS allows absolute quantification of targeted proteins using isotopically labelled peptide standards (AQUA) [30–32] or protein standard absolute quantification (PSAQ) [31,33,34]. Using isotope standards simplifies sample preparation and minimizes variation between mass spectrometry devices, thereby increasing the reliability and accuracy of results.
In the review "Proteomics in Inherited Metabolic Disorders" Maria del Pilar Chantada-Vázquez and collegues describe the application of proteomics methods to the study of Inherited Metabolic Disorders (IMD).
The manuscript is well written and comprehensive, however my suggestion is to either change the title to "Mass Spectrometry (MS) in IMD" or to include in the discussion studies based on Affinity Proteomics as well as MS.
We appreciate the comment. We agree with the reviewer and have included a new subsection in section 2.1 Qualitative and Quantitative Proteomics
2.1.4 Affinity-based (probes and antibodies) proteomics approaches [35–38]
Affinity-based proteomics is a relatively new field of proteomics that seeks to characterize protein activity and protein-protein interaction. This method also allows monitoring of the functional regulation of enzymes in complex proteomes. This technology utilizes small molecule activity-based probes (APBs) or protein antibodies. The probe molecules covalently modify the enzyme active site and allow detection and affinity purification of a target enzyme population. Affinity-based antibodies are mainly generated using microarrays or directly by antibody immunoprecipitation of the whole sample. After affinity purification (pulldown based on probes or antibodies), substrates and their exact cleavage sites are identified by mass spectrometry. Moreover, cleavage sites can be quantitatively evaluated by incorporation of quantitative proteomic techniques such as iTRAQ and SILAC [39]. Thus, this technology is highly selective and can be used for analysis of complex samples such as biological fluids, cell lysates, intact cells, and even whole organisms, and can therefore be applied to the study of IMDs.
All activity-based probes share a similar structure[35,39]. They have elements required for targeting, modification, and detection of labeled proteins. Structurally, those elements can be divided into the reactive group, linker, and tag. The reactive functional group provides a covalent attachment to the catalytic residue of the enzyme active site. The linker function separates the reactive functional group from the tag. Finally, the tag enables visualization and/or purification of labeled proteins.
For the antibody microarray, the data set correlates directly with the number of antibodies included on the array and the range of their specificities [37].
Technologies that reveal the most abundant proteins found in certain samples types (e.g. serum) are crucial for the development of biomarkers that can be used as measurable indicators of disease severity and progression, patient stratification, and drug development. Proximity Extension Assay (PEA) is a novel approach based in the interaction between protein antibodies and DNA [38] that has been commercially developed for the analysis of secreted proteins in serum and blood plasma. This technology can translate protein information into actionable knowledge by linking protein-specific antibodies to DNA-encoded tags. It offers high specificity and sensitivity (sub-pg/ml), enabling high multiplex assays with coverage across a broad dynamic range (~9 log), while consuming minimal quantities of sample. In PEA, matched pairs of oligonucleotide-labeled antibodies bind to their target antigens in a pairwise manner. Upon antibody binding, the matched oligonucleotides are brought into proximity, and using a DNA polymerase a PCR target sequence is created, amplified, detected, and quantified. This downstream process analysis is usually carried out by qPCR or by techniques such as next-generation sequencing (NGS).
The above are all recent technologies, and the coming years will likely see continued growth in the development and application of affinity-based proteomic approaches. The use of probes that target diverse enzyme families has led to a range of applications for activity-based proteomics, in the same way that linking proteins to proteins has provided information about the interactome and protein-DNA interactions have provided further knowledge about cell processes. Therefore, as in other proteomics approaches, comparison of enzyme activity profiles and protein-protein or protein-DNA interactions (based on antibody precipitation) in healthy versus diseased samples can aid the identification of novel biomarkers and drug targets. The results obtained to date show that affinity-based proteomics offer great promise as imaging tools for disease diagnosis and preclinical and clinical testing of therapeutic agents in vivo.
We have also included a new study in table 1 in which the authors used affinity-based methods applied to IMDs, in this case Duchenne muscular dystrophy.
Duchenne muscular dystrophy (DMD; OMIM310200) is a rare X-linked genetic disease with an incidence rate of 1:5,000. DMD is caused by frame-disrupting mutations in the gene encoding dystrophin, resulting in loss of dystrophin [90]. Affected boys typically present clinical signs in the first few years of life, with features suggestive of muscle weakness and often with global developmental delay. Progressive muscle weakness leads to loss of ambulation by the age of 10, and if untreated, to fatal cardio respiratory insufficiency by late adolescence. Establishing a correct diagnosis in dystrophinopathies requires a multidisciplinary approach involving pediatricians, geneticists, and neurologists to define the severity of the clinical phenotype by means of genetic, enzymatic, and immunohistochemical tests [90]. The application of proteomics to the study of dystrophinopathies could facilitate both diagnosis and treatment. In 2014 Ayoglu et al. [36] explored the possibility of identifying circulating candidate protein markers in rare diseases applying an affinity-based proteomics approach to generate a proteomic profile in blood samples from muscular dystrophy patients versus controls. The authors used an antibody bead array platform with 384 antibodies. Based on the results obtained from analysis of both serum and plasma, they identified 11 proteins associated with muscular dystrophy, of which 4 were upregulated in blood from muscular dystrophy patients: carbonic anhydrase III (CA3), myosin light chain 3 (MYL3), mitochondrial malate dehydrogenase 2 (MDH2), and electron transfer flavoprotein A (ETFA). These interesting data could aid the development of new clinical tools for management of dystrophinopathies.
The general description about proteomics methods could be revised cutting (or only mentioning) 2D-PAGE which is a relatively old technique, while including methods such as high multiplexed protein profiling using Proximity Extension Assays (PEA) or Somascan.
A few examples of possibly relevant studies are:
- Cells. 2022 Mar 7;11(5):913. doi: 10.3390/cells11050913.
Hongge Wang et al. Evaluation of Neurofilament Light Chain as a Biomarker of Neurodegeneration in X-Linked Childhood Cerebral Adrenoleukodystrophy
- Blood Adv. 2021 Aug 24;5(16):3092-3101. doi:10.1182/bloodadvances.2020003824.
Brigitte T.A.Long-term effect of hematopoietic cell transplantation on systemic inflammation in patients with mucopolysaccharidoses
We appreciate the reviewer’s comments. We have removed the 2d-PAGE paragraph and included discussion of the PEA technique in section 2.1.4, as referred to in the previous response. The two examples of this technique applied to the study of IMDs were included in table 1 and discussed in the text as we indicated below.
In 2020 van den Broek et al.[71] investigated systemic inflammation in untreated MPS I patients and evaluated the effect of HCT (hematopoietic cell transplantation) on systemic inflammation. The authors used dried blood spot samples from patients with HCT-treated MPS using a OLINK Proseek Multiplex inflammation platform. In total, 92 markers of the OLINK inflammation panel were measured in MPS patients compared with those of age-matched control subjects. The authors observed normal leukocyte enzyme activity levels in 93% of patients post-HCT. Moreover, pretransplant samples showed clear differences between patients and controls. The protein markers that distinguished control subjects from patients pre-HCT were mainly pro-inflammatory (50%) or related to bone homeostasis and extracellular matrix degradation (33%). After 10 years of follow-up, only 5 markers (receptor activator of nuclear factor kappa-B ligand, osteoprotegerin, axis inhibition protein 1 [AXIN1], stem cell factor, and Fms-related tyrosine kinase 3 ligand) remained significantly increased, with a large fold-change difference between MPS I patients and control subjects.
In 2022 Wang et al.[79] used the OLINK Proximity Extension Assay to analyze the cerebral spinal fluid (CSF) of young males with CALD, and performed a comparative analysis using plasma samples. Using the Target 96 Neuro Exploratory panel they found that levels of 5 proteins were significantly increased in CSF. Only for neurofilament light chain (NfL) was a significant correlation observed between CSF and plasma samples. Young males with CALD showed a 11.3-fold increase in plasma NfL compared with controls. Moreover, 9 of 11 young males with CALD who underwent HCT showed a decrease in plasma NfL levels after 1 year of treatment compared with pre-HCT levels. The authors concluded that plasma NfL could constitute a useful determinant of outcomes in CALD
Minor Comments: Figure 1. The sample type should be above the two blocks "Bottom-up" and "Top down" because in the current way it is confusing.
We agree with the reviewer and have edited the figures accordingly.
Figure 4. I would specify the difference between untargeted DIA (eg SWATH) and targeted DIA (SRM/MRM and PRM).
We agree with the reviewer, we change figure 4 adding untargeted DIA for SWATH and targeted DIA for SRM/MRM and PRM. Moreover, the following text has been added to the figure legend:
Figure 4: DDA and DIA mode in proteomic analysis
In DDA mode, extracted proteins are digested and directly analyzed by single-shot DDA. The acquired data is searched against a database of known protein sequences and further processed using diverse software tools. In DIA mode two distinct forms of proteomic analysis can be performed: SWATH-MS (untargeted DIA) and SRM/MRM (targeted DIA). In SWATH-MS extracted proteins are digested and directly analyzed by single-shot analysis in order to generate the spectral library. Once the library is generated a multi-window running method is applied to individual samples: wide isolation windows span the entire MS1 m/z range and all precursor ions in the library that are found in each isolation window are fragmented in MS2. All proteins found in the library are quantified (untargeted) and all samples are processed using data analysis software to obtain the quantitative data. In MRM and SRM, the precursor ions to be analyzed are fixed (targeted) by the user, and only these are fragmented in MS2.
Figure 3 and Figure 4. When talking about targeted MS or quantitative MS the authors should mention that methods such as SRM/MRM and PRM are not simply label free, but they can be quantitative because they imply the use of an internal standard (labeled peptide), while methods based on isobaric or chemical labeling remain semiquantitative.
We agree with the reviewer: it is prudent to emphasize this important difference. The following text has been included at the end of point 2.1.3:
It should be noted that methods such as MRM/SRM/PRM-MS allow for quantification of absolute protein levels. Other previously described methods such as label-based (e.g. ITRAQ, SILAC) and non-label-based methods (e.g. SWATH) are semi quantitative methods that only indicate the fold change in protein levels between two conditions.
Targeted MS/MS allows absolute quantification of targeted proteins using isotopically labelled peptide standards (AQUA) [30–32] or protein standard absolute quantification (PSAQ) [31,33,34]. Using isotope standards simplifies sample preparation and minimizes variation between mass spectrometry devices, thereby increasing the reliability and accuracy of results.
In the review "Proteomics in Inherited Metabolic Disorders" Maria del Pilar Chantada-Vázquez and collegues describe the application of proteomics methods to the study of Inherited Metabolic Disorders (IMD).
The manuscript is well written and comprehensive, however my suggestion is to either change the title to "Mass Spectrometry (MS) in IMD" or to include in the discussion studies based on Affinity Proteomics as well as MS.
We appreciate the comment. We agree with the reviewer and have included a new subsection in section 2.1 Qualitative and Quantitative Proteomics
2.1.4 Affinity-based (probes and antibodies) proteomics approaches [35–38]
Affinity-based proteomics is a relatively new field of proteomics that seeks to characterize protein activity and protein-protein interaction. This method also allows monitoring of the functional regulation of enzymes in complex proteomes. This technology utilizes small molecule activity-based probes (APBs) or protein antibodies. The probe molecules covalently modify the enzyme active site and allow detection and affinity purification of a target enzyme population. Affinity-based antibodies are mainly generated using microarrays or directly by antibody immunoprecipitation of the whole sample. After affinity purification (pulldown based on probes or antibodies), substrates and their exact cleavage sites are identified by mass spectrometry. Moreover, cleavage sites can be quantitatively evaluated by incorporation of quantitative proteomic techniques such as iTRAQ and SILAC [39]. Thus, this technology is highly selective and can be used for analysis of complex samples such as biological fluids, cell lysates, intact cells, and even whole organisms, and can therefore be applied to the study of IMDs.
All activity-based probes share a similar structure[35,39]. They have elements required for targeting, modification, and detection of labeled proteins. Structurally, those elements can be divided into the reactive group, linker, and tag. The reactive functional group provides a covalent attachment to the catalytic residue of the enzyme active site. The linker function separates the reactive functional group from the tag. Finally, the tag enables visualization and/or purification of labeled proteins.
For the antibody microarray, the data set correlates directly with the number of antibodies included on the array and the range of their specificities [37].
Technologies that reveal the most abundant proteins found in certain samples types (e.g. serum) are crucial for the development of biomarkers that can be used as measurable indicators of disease severity and progression, patient stratification, and drug development. Proximity Extension Assay (PEA) is a novel approach based in the interaction between protein antibodies and DNA [38] that has been commercially developed for the analysis of secreted proteins in serum and blood plasma. This technology can translate protein information into actionable knowledge by linking protein-specific antibodies to DNA-encoded tags. It offers high specificity and sensitivity (sub-pg/ml), enabling high multiplex assays with coverage across a broad dynamic range (~9 log), while consuming minimal quantities of sample. In PEA, matched pairs of oligonucleotide-labeled antibodies bind to their target antigens in a pairwise manner. Upon antibody binding, the matched oligonucleotides are brought into proximity, and using a DNA polymerase a PCR target sequence is created, amplified, detected, and quantified. This downstream process analysis is usually carried out by qPCR or by techniques such as next-generation sequencing (NGS).
The above are all recent technologies, and the coming years will likely see continued growth in the development and application of affinity-based proteomic approaches. The use of probes that target diverse enzyme families has led to a range of applications for activity-based proteomics, in the same way that linking proteins to proteins has provided information about the interactome and protein-DNA interactions have provided further knowledge about cell processes. Therefore, as in other proteomics approaches, comparison of enzyme activity profiles and protein-protein or protein-DNA interactions (based on antibody precipitation) in healthy versus diseased samples can aid the identification of novel biomarkers and drug targets. The results obtained to date show that affinity-based proteomics offer great promise as imaging tools for disease diagnosis and preclinical and clinical testing of therapeutic agents in vivo.
We have also included a new study in table 1 in which the authors used affinity-based methods applied to IMDs, in this case Duchenne muscular dystrophy.
Duchenne muscular dystrophy (DMD; OMIM310200) is a rare X-linked genetic disease with an incidence rate of 1:5,000. DMD is caused by frame-disrupting mutations in the gene encoding dystrophin, resulting in loss of dystrophin [90]. Affected boys typically present clinical signs in the first few years of life, with features suggestive of muscle weakness and often with global developmental delay. Progressive muscle weakness leads to loss of ambulation by the age of 10, and if untreated, to fatal cardio respiratory insufficiency by late adolescence. Establishing a correct diagnosis in dystrophinopathies requires a multidisciplinary approach involving pediatricians, geneticists, and neurologists to define the severity of the clinical phenotype by means of genetic, enzymatic, and immunohistochemical tests [90]. The application of proteomics to the study of dystrophinopathies could facilitate both diagnosis and treatment. In 2014 Ayoglu et al. [36] explored the possibility of identifying circulating candidate protein markers in rare diseases applying an affinity-based proteomics approach to generate a proteomic profile in blood samples from muscular dystrophy patients versus controls. The authors used an antibody bead array platform with 384 antibodies. Based on the results obtained from analysis of both serum and plasma, they identified 11 proteins associated with muscular dystrophy, of which 4 were upregulated in blood from muscular dystrophy patients: carbonic anhydrase III (CA3), myosin light chain 3 (MYL3), mitochondrial malate dehydrogenase 2 (MDH2), and electron transfer flavoprotein A (ETFA). These interesting data could aid the development of new clinical tools for management of dystrophinopathies.
The general description about proteomics methods could be revised cutting (or only mentioning) 2D-PAGE which is a relatively old technique, while including methods such as high multiplexed protein profiling using Proximity Extension Assays (PEA) or Somascan.
A few examples of possibly relevant studies are:
- Cells. 2022 Mar 7;11(5):913. doi: 10.3390/cells11050913.
Hongge Wang et al. Evaluation of Neurofilament Light Chain as a Biomarker of Neurodegeneration in X-Linked Childhood Cerebral Adrenoleukodystrophy
- Blood Adv. 2021 Aug 24;5(16):3092-3101. doi:10.1182/bloodadvances.2020003824.
Brigitte T.A.Long-term effect of hematopoietic cell transplantation on systemic inflammation in patients with mucopolysaccharidoses
We appreciate the reviewer’s comments. We have removed the 2d-PAGE paragraph and included discussion of the PEA technique in section 2.1.4, as referred to in the previous response. The two examples of this technique applied to the study of IMDs were included in table 1 and discussed in the text as we indicated below.
In 2020 van den Broek et al.[71] investigated systemic inflammation in untreated MPS I patients and evaluated the effect of HCT (hematopoietic cell transplantation) on systemic inflammation. The authors used dried blood spot samples from patients with HCT-treated MPS using a OLINK Proseek Multiplex inflammation platform. In total, 92 markers of the OLINK inflammation panel were measured in MPS patients compared with those of age-matched control subjects. The authors observed normal leukocyte enzyme activity levels in 93% of patients post-HCT. Moreover, pretransplant samples showed clear differences between patients and controls. The protein markers that distinguished control subjects from patients pre-HCT were mainly pro-inflammatory (50%) or related to bone homeostasis and extracellular matrix degradation (33%). After 10 years of follow-up, only 5 markers (receptor activator of nuclear factor kappa-B ligand, osteoprotegerin, axis inhibition protein 1 [AXIN1], stem cell factor, and Fms-related tyrosine kinase 3 ligand) remained significantly increased, with a large fold-change difference between MPS I patients and control subjects.
In 2022 Wang et al.[79] used the OLINK Proximity Extension Assay to analyze the cerebral spinal fluid (CSF) of young males with CALD, and performed a comparative analysis using plasma samples. Using the Target 96 Neuro Exploratory panel they found that levels of 5 proteins were significantly increased in CSF. Only for neurofilament light chain (NfL) was a significant correlation observed between CSF and plasma samples. Young males with CALD showed a 11.3-fold increase in plasma NfL compared with controls. Moreover, 9 of 11 young males with CALD who underwent HCT showed a decrease in plasma NfL levels after 1 year of treatment compared with pre-HCT levels. The authors concluded that plasma NfL could constitute a useful determinant of outcomes in CALD
Minor Comments: Figure 1. The sample type should be above the two blocks "Bottom-up" and "Top down" because in the current way it is confusing.
We agree with the reviewer and have edited the figures accordingly.
Figure 4. I would specify the difference between untargeted DIA (eg SWATH) and targeted DIA (SRM/MRM and PRM).
We agree with the reviewer, we change figure 4 adding untargeted DIA for SWATH and targeted DIA for SRM/MRM and PRM. Moreover, the following text has been added to the figure legend:
Figure 4: DDA and DIA mode in proteomic analysis
In DDA mode, extracted proteins are digested and directly analyzed by single-shot DDA. The acquired data is searched against a database of known protein sequences and further processed using diverse software tools. In DIA mode two distinct forms of proteomic analysis can be performed: SWATH-MS (untargeted DIA) and SRM/MRM (targeted DIA). In SWATH-MS extracted proteins are digested and directly analyzed by single-shot analysis in order to generate the spectral library. Once the library is generated a multi-window running method is applied to individual samples: wide isolation windows span the entire MS1 m/z range and all precursor ions in the library that are found in each isolation window are fragmented in MS2. All proteins found in the library are quantified (untargeted) and all samples are processed using data analysis software to obtain the quantitative data. In MRM and SRM, the precursor ions to be analyzed are fixed (targeted) by the user, and only these are fragmented in MS2.
Figure 3 and Figure 4. When talking about targeted MS or quantitative MS the authors should mention that methods such as SRM/MRM and PRM are not simply label free, but they can be quantitative because they imply the use of an internal standard (labeled peptide), while methods based on isobaric or chemical labeling remain semiquantitative.
We agree with the reviewer: it is prudent to emphasize this important difference. The following text has been included at the end of point 2.1.3:
It should be noted that methods such as MRM/SRM/PRM-MS allow for quantification of absolute protein levels. Other previously described methods such as label-based (e.g. ITRAQ, SILAC) and non-label-based methods (e.g. SWATH) are semi quantitative methods that only indicate the fold change in protein levels between two conditions.
Targeted MS/MS allows absolute quantification of targeted proteins using isotopically labelled peptide standards (AQUA) [30–32] or protein standard absolute quantification (PSAQ) [31,33,34]. Using isotope standards simplifies sample preparation and minimizes variation between mass spectrometry devices, thereby increasing the reliability and accuracy of results.
Author Response File: Author Response.pdf