Synthesis and Structure-Activity Relationships of (−)-cis-N-Normetazocine-Based LP1 Derivatives
Abstract
: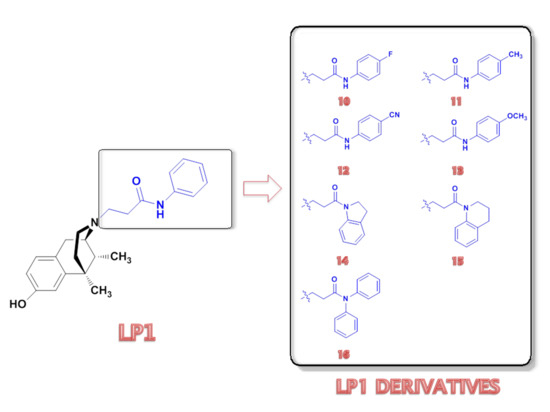
1. Introduction
2. Results and Discussion
2.1. Chemistry
2.2. Radioligand Binding Assay
2.3. Calcium Mobilization Assay
3. Materials and Methods
3.1. Chemistry
3.1.1. General Experimental Procedures
3.1.2. General Procedure for the Preparation of 3-Bromo Propanamide Derivatives (3–9)
3.1.3. General Procedure for the Synthesis of N-Substituted (−)-Cis-N-Normetazocine Derivatives (10–16)
3.2. Biological Assays
3.2.1. Drugs and Reagents
3.2.2. Cell Membrane Preparations
3.2.3. In Vitro Competition Radioligand Binding Assay
3.2.4. Transfection Procedures and Cell Culture
3.2.5. Cell Culture and Calcium Mobilization Experiments
3.2.6. Data Analysis
4. Conclusions
Author Contributions
Acknowledgments
Conflicts of Interest
References
- Janecka, A.; Fichna, J.; Janecki, T. Opioid receptors and their ligands. Curr. Top. Med. Chem. 2004, 4, 1–17. [Google Scholar] [CrossRef] [PubMed]
- Turnaturi, R.; Aricò, G.; Ronsisvalle, G.; Parenti, C.; Pasquinucci, L. Multitarget opioid ligands in pain relief: New players in an old game. Eur. J. Med. Chem. 2016, 108, 211–228. [Google Scholar] [CrossRef] [PubMed]
- Turnaturi, R.; Aricò, G.; Ronsisvalle, G.; Pasquinucci, L.; Parenti, C. Multitarget Opioid/Non-opioid Ligands: A Potential Approach in Pain Management. Curr. Med. Chem. 2016, 23, 4506–4528. [Google Scholar] [CrossRef] [PubMed]
- Turnaturi, R.; Pasquinucci, L.; Parenti, C. From Plant to Bench: Natural Products as Source for Analgesic Drug Development. Front. Nat. Prod. Chem. 2018. submitted for publication. [Google Scholar]
- Pathan, H.; Williams, J. Basic opioid pharmacology: An update. Br. J. Pain 2012, 6, 11–16. [Google Scholar] [CrossRef] [PubMed]
- Ghelardini, C.; Di Cesare Mannelli, L.; Bianchi, E. The pharmacological basis of opioids. Clin. Cases Miner. Bone Metab. 2015, 12, 219–221. [Google Scholar] [CrossRef] [PubMed]
- Zadina, J.E.; Martin-Schild, S.; Gerall, A.A.; Kastin, A.J.; Hackler, L.; Ge, L.J.; Zhang, X. Endomorphins: Novel endogenous mu-opiate receptor agonists in regions of high mu-opiate receptor density. Ann. N. Y. Acad. Sci. 1999, 897, 136–144. [Google Scholar] [CrossRef] [PubMed]
- Shulgin, A.T. Drugs of abuse in the future. Clin. Toxicol. 1975, 8, 405–456. [Google Scholar] [CrossRef] [PubMed]
- Pasquinucci, L.; Iadanza, M.; Marrazzo, A.; Prezzavento, O.; Ronsisvalle, S.; Scoto, G.M.; Parenti, C.; De Luca, L.; Ronsisvalle, G. New benzomorphan derivatives of MPCB as MOP and KOP receptor ligands. Pharmazie 2007, 62, 813–824. [Google Scholar] [CrossRef] [PubMed]
- Metcalf, M.D.; Aceto, M.D.; Harris, L.S.; Woods, J.H.; Traynor, J.R.; Coop, A.; May, E.L. The influence of esters and carboxylic acids as the N-substituent of opioids. Part 1: Benzomorphans. Bioorg. Med. Chem. 2008, 16, 869–873. [Google Scholar] [CrossRef] [PubMed]
- May, E.L.; Jacobson, A.E.; Mattson, M.V.; Traynor, J.R.; Woods, J.H.; Harris, L.S.; Bowman, E.R.; Aceto, M.D. Synthesis and in vitro and in vivo activity of (−)-(1R,5R,9R)- and (+)-(1S,5S,9S)-N-Alkenyl-, -N-Alkynyl-, and -N-Cyanoalkyl-5, 9-dimethyl-2’-hydroxy-6,7-benzomorphan homologues. J. Med. Chem. 2000, 43, 5030–5036. [Google Scholar] [CrossRef] [PubMed]
- Turnaturi, R.; Marrazzo, A.; Parenti, C.; Pasquinucci, L. Benzomorphan scaffold for opioid analgesics and pharmacological tools development: A comprehensive review. Eur. J. Med. Chem. 2018, 148, 410–422. [Google Scholar] [CrossRef] [PubMed]
- Dortch-Carnes, J.; Potter, D.E. Bremazocine: A kappa-opioid agonist with potent analgesic and other pharmacologic properties. CNS Drug Rev. 2005, 11, 195–212. [Google Scholar] [CrossRef] [PubMed]
- Pasquinucci, L.; Prezzavento, O.; Marrazzo, A.; Amata, E.; Ronsisvalle, S.; Georgoussi, Z.; Fourla, D.D.; Scoto, G.M.; Parenti, C.; Aricò, G.; et al. Evaluation of N-substitution in 6,7-benzomorphan compounds. Bioorg. Med. Chem. 2010, 18, 4975–4982. [Google Scholar] [CrossRef] [PubMed]
- Parenti, C.; Turnaturi, R.; Aricò, G.; Marrazzo, A.; Prezzavento, O.; Ronsisvalle, S.; Scoto, G.M.; Ronsisvalle, G.; Pasquinucci, L. Antinociceptive profile of LP1, a non-peptide multitarget opioid ligand. Life Sci. 2012, 90, 957–961. [Google Scholar] [CrossRef] [PubMed]
- Pasquinucci, L.; Parenti, C.; Turnaturi, R.; Aricò, G.; Marrazzo, A.; Prezzavento, O.; Ronsisvalle, S.; Georgoussi, Z.; Fourla, D.D.; Scoto, G.M.; et al. The benzomorphan-based LP1 ligand is a suitable MOR/DOR agonist for chronic pain treatment. Life Sci. 2012, 90, 66–70. [Google Scholar] [CrossRef] [PubMed]
- Parenti, C.; Turnaturi, R.; Aricò, G.; Gramowski-Voss, A.; Schroeder, O.H.; Marrazzo, A.; Prezzavento, O.; Ronsisvalle, S.; Scoto, G.M.; Ronsisvalle, G.; et al. The multitarget opioid ligand LP1's effects in persistent pain and in primary cell neuronal cultures. Neuropharmacology 2013, 71, 70–82. [Google Scholar] [CrossRef] [PubMed]
- Pasquinucci, L.; Turnaturi, R.; Aricò, G.; Parenti, C.; Pallaki, P.; Georgoussi, Z.; Ronsisvalle, S. Evaluation of N-substituent structural variations in opioid receptor profile of LP1. Bioorg. Med. Chem. 2016, 24, 2832–2842. [Google Scholar] [CrossRef] [PubMed]
- Turnaturi, R.; Parenti, C.; Prezzavento, O.; Marrazzo, A.; Pallaki, P.; Georgoussi, Z.; Amata, E.; Pasquinucci, L. Synthesis and Structure-Activity Relationships of LP1 Derivatives: N-Methyl-N-phenylethylamino Analogues as Novel MOR Agonists. Molecules 2018, 23, E677. [Google Scholar] [CrossRef] [PubMed]
- Pasquinucci, L.; Turnaturi, R.; Prezzavento, O.; Arena, E.; Aricò, G.; Georgoussi, Z.; Parenti, R.; Cantarella, G.; Parenti, C. Development of novel LP1-based analogues with enhanced delta opioid receptor profile. Bioorg. Med. Chem. 2017, 25, 4745–4752. [Google Scholar] [CrossRef] [PubMed]
- Accolla, M.L.; Turnaturi, R.; Sarpietro, M.G.; Ronsisvalle, S.; Castelli, F.; Pasquinucci, L. Differential scanning calorimetry approach to investigate the transfer of the multitarget opioid analgesic LP1 to biomembrane model. Eur. J. Med. Chem. 2014, 77, 84–90. [Google Scholar] [CrossRef] [PubMed]
- Vandormael, B.; Fourla, D.D.; Gramowski-Voss, A.; Kosson, P.; Weiss, D.G.; Schröder, O.H.; Lipkowski, A.; Georgoussi, Z.; Tourwé, D. Superpotent [Dmt¹] dermorphin tetrapeptides containing the 4-aminotetrahydro-2-benzazepin-3-one scaffold with mixed μ/δ opioid receptor agonistic properties. J. Med. Chem. 2011, 54, 7848–7859. [Google Scholar] [CrossRef] [PubMed]
- Papakonstantinou, M.P.; Karoussiotis, C.; Georgoussi, Z. RGS2 and RGS4 proteins: New modulators of the κ-opioid receptor signaling. Cell Signal. 2015, 27, 104–114. [Google Scholar] [CrossRef] [PubMed]
- Camarda, V.; Calò, G. Chimeric G proteins in fluorimetric calcium assays: Experience with opioid receptors. Methods Mol. Biol. 2013, 937, 293–306. [Google Scholar] [CrossRef] [PubMed]
- Georgoussi, Z.; Zioudrou, C. Effect of delta-opioid antagonists on the functional coupling between opioid receptors and G-proteins in rat brain membranes. Biochem. Pharmacol. 1993, 45, 2405–2410. [Google Scholar] [CrossRef]
- Guerrini, R.; Calò, G.; Rizzi, A.; Bianchi, C.; Lazarus, L.H.; Salvadori, S.; Temussi, P.A.; Regoli, D. Address and message sequences for the nociceptin receptor: A structure-activity study of nociceptin-(1-13)-peptide amide. J. Med. Chem. 1997, 40, 1789–1793. [Google Scholar] [CrossRef] [PubMed]
- Bradford, M.M. A rapid and sensitive method for the quantitation of microgram quantities of protein utilizing the principle of protein-dye binding. Anal. Biochem. 1976, 72, 248–254. [Google Scholar] [CrossRef]
- Prezzavento, O.; Arena, E.; Parenti, C.; Pasquinucci, L.; Aricò, G.; Scoto, G.M.; Grancara, S.; Toninello, A.; Ronsisvalle, S. Design and synthesis of new bifunctional sigma-1 selective ligands with antioxidant activity. J. Med. Chem. 2013, 56, 2447–2455. [Google Scholar] [CrossRef] [PubMed]
- Georgoussi, Z.; Merkouris, M.; Mullaney, I.; Megaritis, G.; Carr, G.; Zioudrou, C.; Milligan, G. Selective interactions of the μ-opioid receptors with pertussis toxin-sensitive G proteins: Involvement of the third intracellular loop and the C-terminal tail in coupling. Biochem. Biophys. Acta 1997, 1359, 263–274. [Google Scholar] [CrossRef]
- Morou, E.; Georgoussi, Z. Expression of the third intracellular loop of the delta-opioid receptor inhibits signaling by opioid receptors and other G protein-coupled receptors. J. Pharmacol. Exp. Ther. 2005, 315, 1368–1379. [Google Scholar] [CrossRef] [PubMed]
Compound | Ki (μM) ± SEM a,b | ||
---|---|---|---|
MOR | DOR | KOR | |
LP1 (1) | 0.049 ± 0.015 | 0.033 ± 0.020 | 7.500 ± 0.015 |
2 [18] | 0.038±0.004 | 0.210 ± 0.030 | 0.800 ± 0.016 |
10 | 0.280 ± 0.013 | 0.920 ± 0.120 | 4.800 ± 0.950 |
11 | 0.240 ± 0.015 | 0.410 ± 0.023 | 1.010 ± 0.110 |
12 | 0.210 ± 0.024 | 0.380 ± 0.034 | 0.850 ± 0.180 |
13 | 0.180 ± 0.011 | 1.100 ± 0.110 | 4.000 ± 0.180 |
14 | 0.400 ± 0.030 | 1.400 ± 0.120 | 1.600 ± 0.120 |
15 | 0.360 ± 0.023 | NDc | 0.200 ± 0.020 |
16 | 0.170 ± 0.013 | ND | 0.200 ± 0.030 |
Compound | MOR | DOR | KOR | |||
---|---|---|---|---|---|---|
pEC50 (CL95%) a | pKB b (CL95%) | pEC50 (CL95%) a | pKB b (CL95%) | pEC50 (CL95%) a | pKB b (CL95%) | |
LP1 (1) | 7.01 (6.29–7.73) | ND c | 5.95 (5.39–6.51) | ND | crc incomplete d | ND |
2 | Inactive e | 7.90 (7.30–8.50) | Inactive | 6.78 (6.15–7.41) | Inactive | 5.86 (4.89–6.83) |
10 | 5.67 (4.82–6.52) | ND | crc incomplete | Inactive | crc incomplete | ND |
11 | 5.85 (5.34–6.36) | ND | Inactive | 5.83 (5.53–6.13) | Inactive | 5.85 (5.65–6.05) |
12 | 5.99 (5.46–6.52) | ND | Inactive | 5.82 (5.18–6.46) | Inactive | 5.90 (5.40–6.40) |
13 | Inactive | 6.30 (5.81–6.79) | Inactive | Inactive | Inactive | Inactive |
14 | Inactive | 5.94 (5.26–6.62) | Inactive | Inactive | Inactive | Inactive |
15 | Inactive | 5.71 (4.63–6.18) | Inactive | Inactive | 6.59 (6.35–6.83) | ND |
16 | Inactive | 6.12 (5.46–6.78) | Inactive | Inactive | Inactive | 6.11 (5.78–6.44) |
© 2018 by the authors. Licensee MDPI, Basel, Switzerland. This article is an open access article distributed under the terms and conditions of the Creative Commons Attribution (CC BY) license (http://creativecommons.org/licenses/by/4.0/).
Share and Cite
Pasquinucci, L.; Parenti, C.; Amata, E.; Georgoussi, Z.; Pallaki, P.; Camarda, V.; Calò, G.; Arena, E.; Montenegro, L.; Turnaturi, R. Synthesis and Structure-Activity Relationships of (−)-cis-N-Normetazocine-Based LP1 Derivatives. Pharmaceuticals 2018, 11, 40. https://doi.org/10.3390/ph11020040
Pasquinucci L, Parenti C, Amata E, Georgoussi Z, Pallaki P, Camarda V, Calò G, Arena E, Montenegro L, Turnaturi R. Synthesis and Structure-Activity Relationships of (−)-cis-N-Normetazocine-Based LP1 Derivatives. Pharmaceuticals. 2018; 11(2):40. https://doi.org/10.3390/ph11020040
Chicago/Turabian StylePasquinucci, Lorella, Carmela Parenti, Emanuele Amata, Zafiroula Georgoussi, Paschalina Pallaki, Valeria Camarda, Girolamo Calò, Emanuela Arena, Lucia Montenegro, and Rita Turnaturi. 2018. "Synthesis and Structure-Activity Relationships of (−)-cis-N-Normetazocine-Based LP1 Derivatives" Pharmaceuticals 11, no. 2: 40. https://doi.org/10.3390/ph11020040
APA StylePasquinucci, L., Parenti, C., Amata, E., Georgoussi, Z., Pallaki, P., Camarda, V., Calò, G., Arena, E., Montenegro, L., & Turnaturi, R. (2018). Synthesis and Structure-Activity Relationships of (−)-cis-N-Normetazocine-Based LP1 Derivatives. Pharmaceuticals, 11(2), 40. https://doi.org/10.3390/ph11020040